Europe PMC requires Javascript to function effectively.
Either your web browser doesn't support Javascript or it is currently turned off. In the latter case, please turn on Javascript support in your web browser and reload this page.

PDX Mouse Models Are Reliable Stand-Ins for Human Tumors, Study Finds
February 2, 2021 , by Carmen Phillips
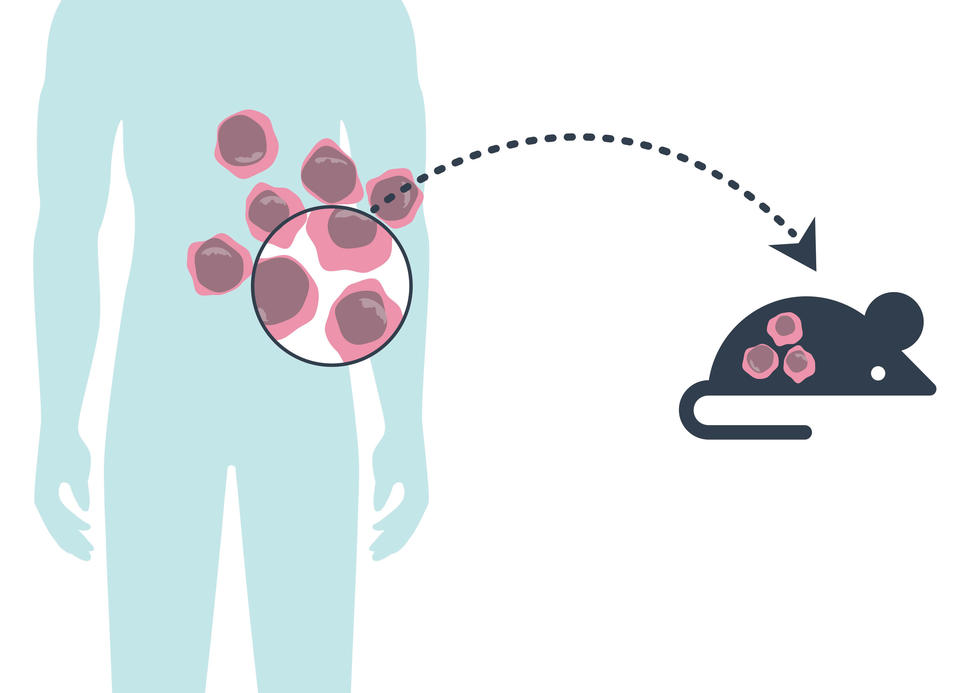
PDX mice largely retain the genetics of the human tumors from which they were initially created, a new study shows.
Mouse models are among the most valuable tools in cancer research. Researchers use them for many types of studies, from identifying possible new cancer treatments to finding new clues about cancer biology.
But mice aren’t humans. So developing mouse models that provide meaningful research results—namely, by ensuring they replicate how cancer behaves in humans as closely as possible—has been a high priority for the research community. Now, a large study from an international group of researchers has provided some strong reassurance that an increasingly relied-upon type of mouse model , known as PDX mice, does just that.
PDX is shorthand for patient-derived xenograft , meaning that the models are initially created by implanting a fragment of a human tumor into a mouse. And this new study shows that the resulting population of these PDX mice largely retain the genetics of the human tumors from which they were initially created . There was also evidence that few substantial changes developed in cancer-related genes in the mouse tumors, the researchers reported January 7 in Nature Genetics .
Coming from the most comprehensive study of its kind done to date, the findings should give researchers confidence about the validity of studies using PDX models, said one of the study’s lead investigators, Jeffrey Chuang, Ph.D., of the Jackson Laboratory for Genomic Medicine.
That’s incredibly important, because results from such studies are increasingly being used to decide whether to move experimental treatments forward into human clinical trials, Dr. Chuang explained. “To get to the clinical trial stage is such a big investment, so you want the best data possible” to make those decisions, he said.
Not surprisingly, the study did show that there is some variation between human tumors and the versions of those tumors that are present in PDX mice, explained Senthil Muthuswamy, Ph.D., of Beth Israel Deaconess Medical Center and Harvard Medical School, who develops research models of cancer but was not involved in this study.
But that’s not surprising, Dr. Muthuswamy continued. “No model is perfect. Just because a model is not a perfect reflection of human tumors, that doesn’t mean it’s not useful,” he continued. “There are previous studies showing that the PDX models have very good utility, and this study conveys that message quite robustly.”
Cancer Research in a “Biological System”
Animal models are essential to some cancer researchers because they allow them to study a tumor in a more biologically relevant manner than is possible with other models, such as cancer cells in a laboratory dish, Dr. Chuang explained.
A tumor in an animal model “is in a growing tissue,” he said. “It has the same characteristics as [human] tumors. There are blood vessels in and around the tumor, there is interaction with” the cells and tissue around the tumor.
For a number of reasons, both biologic and economic, mice are the most commonly used animal model. There are several kinds of mouse models. The most commonly used are those created by injecting mice with cancer cells that have been grown and maintained in laboratory dishes (known as cell lines ). That’s because these cells reliably and quickly grow into tumors, providing a relatively inexpensive and easy way to rapidly produce a large number of mice to study.
Although they are an important tool, these models also have important limitations, explained Jeffrey Moscow, M.D., of the Cancer Therapy Evaluation Program in NCI’s Division of Cancer Treatment and Diagnosis, an investigator on the study.
For example, because cancer cell lines are stored and grown in artificial conditions, they tend to undergo numerous genomic changes over time, Dr. Moscow said, meaning they can’t necessarily provide “a faithful representation of a patient’s tumor.”
Making a Better Mouse Model
PDX models were developed to help address the shortcomings of other available models. The mice used for PDX models are bred to lack an immune system , which protects the implanted tumor fragment from being attacked and destroyed by the animals’ immune cells.
Once the original human tumor fragment has taken, or engrafted, in a mouse and grows into a full-blown tumor, that original animal is then scaled up. That’s done by taking fragments of its tumor and implanting into several more mice—a process known as passaging.
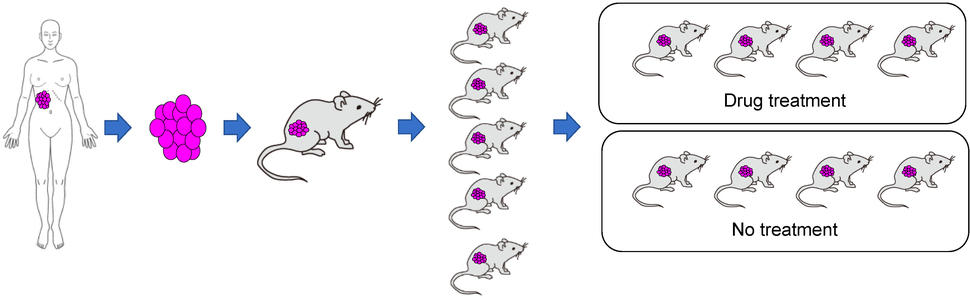
PDX mouse models of cancer are used for different types of studies, including those that help determine whether experimental therapies should be tested in human clinical trials.
Once the tumor fragments in those mice have grown into full-blown tumors, further passaging rounds are done until enough of the mice are available to perform a given study. In contrast to models produced with cell lines, creating a PDX model can be a time-consuming process.
“To fully establish a model can take from 6 months to a year,” Dr. Chuang said.
Despite the greater time and cost involved, PDX models have emerged as a research workhorse, particularly for studies involving experimental drugs or drug combinations, Dr. Moscow explained. At NCI, he said, results from PDX model studies “are one of the major criteria we use to prioritize which [drugs] we’re going to move into clinical trials.”
Addressing the Big Question about PDX Models
Recently, questions have been raised about the wisdom of the growing reliance on PDX models.
A chief concern is whether, as the original bit of human tumor travels through multiple rounds of passaging, the evolutionary pressures put on them as they grow in mouse after mouse might cause the tumors to undergo genomic changes, including different changes than those that occur in humans. If there is enough divergence from the original tumor, studies performed in these mice might not reflect what would happen in a human.
A 2017 study from researchers at Harvard and Massachusetts Institute of Technology (MIT) appeared to confirm those fears. The research team found that tumors in PDX mice quickly developed genetic changes known as copy number alterations , in which there are more or less than two copies of particular genes.
The changes arose within a few rounds of passaging, they reported, and differed from the genomic changes seen in the human tumors they analyzed. There was also evidence that the changes seen in the mouse tumors affected how they responded to cancer therapies.
But that study had some important limitations, Dr. Moscow said, in particular that it used an RNA -based method to measure gene expression . This method may not reliably capture changes like copy number alterations in PDX mice, he continued, because the comparisons between the original human tumor and the PDX models can be muddled by the presence of bits of normal human tissue in the original tumor sample.
Meanwhile, several smaller studies reached the opposite conclusion, showing strong parallels between tumors in PDX mice and the human tumors from which they were established.
What was needed was a study to resolve these “contradictory observations,” Dr. Chuang and his colleagues wrote.
A More Comprehensive Analysis of PDX Mice
The new study was conducted by researchers from two large consortia focused on advancing the use and science of PDX models: the NCI Cancer Moonshot–funded PDXNet and its European counterpart, EurOPDX.
To conduct the study, the researchers analyzed samples from more than 500 PDX models and more than 300 matched samples of human tumors. The study covered 16 cancer types and tumors from patients in North America, Europe, and Asia.
In addition to assessing copy number alterations in the PDX mice using the RNA-based technique used in the Harvard/MIT study, this new and larger study went several steps further, employing several DNA -based methods to detect genetic changes, including whole-genome and whole-exome sequencing.
This more comprehensive and collaborative approach allowed the researchers “to more accurately assess genetic changes over time by using many analytic techniques and PDX models from many different laboratories,” Dr. Moscow said.
Providing Confidence on PDX Models
The result was that they did not find extensive copy number alterations between human tumors and those in the PDX models. This was the case even in “late-passage” models—that is, in mice many times removed from the original PDX mouse.
Even when the researchers looked specifically at a trio of matched samples—tumor samples from patients with metastatic colorectal and breast cancer and from their respective early-passage and late-passage models—they largely resembled each other.
Overall, Dr. Chuang said, the study should provide confidence that genomic changes in PDX models “are not a problem.”
Dr. Muthuswamy praised the two groups for conducting the study, stressing that it will help move the science of cancer models forward.
His laboratory develops three-dimensional models called organoids, which also use cancer cells taken directly from patients’ tumors that are induced to grow into “mini-organs” in the laboratory. Unlike cancer cells in a dish, these models can replicate some aspects of how tumors develop in human tissue and maintain the genetic makeup and tissue structure of the original tumors, but they can be developed more rapidly and cheaply than PDX mice.
He believes that organoid models can eventually progress to the point where they can be used as a way to prioritize the most promising drugs to test in PDX models.
Overall, Dr. Muthuswamy said that he’s hopeful that more researchers will begin to use PDX models. Thanks to programs like NCI’s Patient-Derived Models Repository , “the availability of PDX models is much better now,” he said. “These are the next phase of cancer models that we need to use, because they capture some level of patient-to-patient variation. They’re really very important.”
Featured Posts
August 22, 2024, by Carmen Phillips
July 24, 2024, by Sharon Reynolds
July 9, 2024, by Linda Wang
- Biology of Cancer
- Cancer Risk
- Childhood Cancer
- Clinical Trial Results
- Disparities
- FDA Approvals
- Global Health
- Leadership & Expert Views
- Screening & Early Detection
- Survivorship & Supportive Care
- September (5)
- February (6)
- January (6)
- December (7)
- November (6)
- October (7)
- September (7)
- February (7)
- November (7)
- October (5)
- September (6)
- November (4)
- September (9)
- February (5)
- October (8)
- January (7)
- December (6)
- September (8)
- February (9)
- December (9)
- November (9)
- October (9)
- September (11)
- February (11)
- January (10)
- DOI: 10.1038/nri3129
- Corpus ID: 205491533
Experimental mouse tumour models: what can be learnt about human cancer immunology?
- Published in Nature reviews. Immunology 2 December 2011
- Medicine, Biology
121 Citations
Animal models for translational cancer immunotherapy studies, mouse models of tumor immunotherapy., genetically engineered mouse models of cancer reveal new insights about the antitumor immune response., toward the clinical development of synthetic immunity to cancer.
- Highly Influenced
Late-stage MC38 tumours recapitulate features of human colorectal cancer – implications for appropriate timepoint selection in preclinical studies
Improved mouse models to assess tumour immunity and iraes after combination cancer immunotherapies, top 10 challenges in cancer immunotherapy., preclinical vaccines against mammary carcinoma, orientation of preclinical research in ovarian cancer, neutrophils: critical components in experimental animal models of cancer., 61 references, animal models of tumor immunity, immunotherapy and cancer vaccines., natural innate and adaptive immunity to cancer., cancer immunoediting: integrating immunity’s roles in cancer suppression and promotion, gsk's antigen-specific cancer immunotherapy programme: pilot results leading to phase iii clinical development., vaccination for treatment and prevention of cancer in animal models., dual roles for immunity in gastrointestinal cancers., immunosuppressive strategies that are mediated by tumor cells., determinants of successful cd8+ t-cell adoptive immunotherapy for large established tumors in mice, eradication of established tumors by cd8+ t cell adoptive immunotherapy., combination immunotherapy of primary prostate cancer in a transgenic mouse model using ctla-4 blockade., related papers.
Showing 1 through 3 of 0 Related Papers
Experimental mouse tumour models: What can be learnt about human cancer immunology?
- December 2011
- Nature Reviews Immunology 12(1):61-6
- This person is not on ResearchGate, or hasn't claimed this research yet.
Discover the world's research
- 25+ million members
- 160+ million publication pages
- 2.3+ billion citations
No full-text available

To read the full-text of this research, you can request a copy directly from the author.
- INT J CANCER

- Livia De Paolis

- IMMUNOL REV
- Julie Garcia
- Cassandra E. Burnett

- Briséis Varin

- CANCER METAST REV
- Michael W. Lee

- Chuan-Wei Jang
- Tsung-Lin Tsai
- Jinghui Xiu

- Jillian R. Love
- Wouter R. Karthaus
- Qiuliang Li
- Mônica Horr

- Martin W. LaFleur
- Arlene H. Sharpe
- Koji Terada

- Hirohito Ishigaki
- Marcus A. C. Williams
- Cooper Wiens
- Adam Hamilton

- NAT REV IMMUNOL

- Pandian Sureshbabu Ram Kumar

- ADV DRUG DELIVER REV

- CELL DEATH DIFFER

- Takako Hirata
- CANCER IMMUNOL IMMUN

- Priti S. Hegde
- Daniel S. Chen

- Monique E Verhaegen
- Mallory Joseph

- RADIOTHER ONCOL
- Si-Wei Wang
- Qiu-Yi Zheng
- Wei-Feng Hong

- EXPERT OPIN THER TAR

- Xiangna Guan
- Xuebin Liao
- Hamad Ghaleb Dailah
- Abdullah Abdu Hommdi
- Mahdi Dafer Koriri

- Ranxi Liang

- Xijian Chen
- Hsuan Ping Chang
- Yuen Kiu Cheung

- Megha Karne

- Vandana S. Nikam
- ASIA-PAC J CLIN ONCO

- Estelle M. Peyroux
- Angela L. Ferguson

- Rich Woessner
- Alexandra Borodovsky

- Xiaobo Chen
- Yanghui Sheng

- Silvana Azevedo

- Yu Chi Yang

- EUR J IMMUNOL

- BREAST CANCER RES TR

- Chiara Gorrini
- David W. Cescon
- A. Blanchard

- David Adler
- Frank Kramer

- Lionel Apetoh
- Fran|[ccedil]|ois Ghiringhelli

- Laurence Zitvogel
- Sci Transl Med

- P NATL ACAD SCI USA
- Brian Ruffell

- Robert Moore
- Richard G Thompson
- Frances Balkwill

- Jeffrey W. Pollard
- NEW ENGL J MED

- Jedd D. Wolchok
- J CLIN INVEST

- Donna Neuberg
- Michael Dougan
- Glenn Dranoff

- ANNU REV IMMUNOL

- Michael H Kershaw
- Robert D Schreiber
- Mark J Smyth

- Akgül Akpinarli

- J CLIN ONCOL
- T. J. Schuetz
- Brent A. Blumenstein

- Mohit Aggarwal
- Santiago Montes-Moreno

- Michael C. Milone
- Raffit Hassan

- Derek Ng Tang
- Padmanee Sharma

- Richard C. Mulligan

- Andrea van Elsas

- Stefania Rovero

- Guido Forni
- R Todd Reilly

- Jose R. Conejo-Garcia

- Marc R Theoret

- Anne M Ercolini

- Elizabeth A Manning

- Karl S Peggs

- Alberto Mantovani
- Paola Allavena

- Lloyd J. Old
- Michel J. DuPage
- Ann F Cheung
- Claire Mazumdar
- Tyler Jacks

- Samantha Solito
- Vincenzo Bronte
- F. Stephen Hodi

- David F. McDermott
- Walter J. Urba

- Ira Wollner
- Suzanne L. Topalian

- Michael Karin

- Eric J Small

- Ivan Martinez-Forero

- Lieping Chen

- Melinda VanRoey
- Changyu Wang

- A. and Van Pel

- Holly L Hanson

- Hiroaki Ikeda

- Donald A. Clarke
- Baruj Benacerraf

- Jeff F. Miller

- ADV IMMUNOL
- Alan J. Korman

- Rienk Offringa

- Recruit researchers
- Join for free
- Login Email Tip: Most researchers use their institutional email address as their ResearchGate login Password Forgot password? Keep me logged in Log in or Continue with Google Welcome back! Please log in. Email · Hint Tip: Most researchers use their institutional email address as their ResearchGate login Password Forgot password? Keep me logged in Log in or Continue with Google No account? Sign up

An official website of the United States government
The .gov means it’s official. Federal government websites often end in .gov or .mil. Before sharing sensitive information, make sure you’re on a federal government site.
The site is secure. The https:// ensures that you are connecting to the official website and that any information you provide is encrypted and transmitted securely.
- Publications
- Account settings
- My Bibliography
- Collections
- Citation manager
Save citation to file
Email citation, add to collections.
- Create a new collection
- Add to an existing collection
Add to My Bibliography
Your saved search, create a file for external citation management software, your rss feed.
- Search in PubMed
- Search in NLM Catalog
- Add to Search
Experimental mouse models for translational human cancer research
Affiliation.
- 1 Department of Thoracic Surgery, The Second Affiliated Hospital, Air Force Medicel University, Xi'an, China.
- PMID: 36969176
- PMCID: PMC10036357
- DOI: 10.3389/fimmu.2023.1095388
The development and growth of tumors remains an important and ongoing threat to human life around the world. While advanced therapeutic strategies such as immune checkpoint therapy and CAR-T have achieved astonishing progress in the treatment of both solid and hematological malignancies, the malignant initiation and progression of cancer remains a controversial issue, and further research is urgently required. The experimental animal model not only has great advantages in simulating the occurrence, development, and malignant transformation mechanisms of tumors, but also can be used to evaluate the therapeutic effects of a diverse array of clinical interventions, gradually becoming an indispensable method for cancer research. In this paper, we have reviewed recent research progress in relation to mouse and rat models, focusing on spontaneous, induced, transgenic, and transplantable tumor models, to help guide the future study of malignant mechanisms and tumor prevention.
Keywords: PDX; animal model; immune microenvironment; immunodeficiency; induced; spontaneity; transgene; tumor.
Copyright © 2023 Zhou, Xia, Xu, She, Zhang, Sun, Wen, Jiang, Xiong and Lei.
PubMed Disclaimer
Conflict of interest statement
The authors declare that the research was conducted in the absence of any commercial or financial relationships that could be construed as a potential conflict of interest.
The incidence of various spontaneous…
The incidence of various spontaneous tumors in different strains of mice (rat).
Different types of compounds can…
Different types of compounds can induce tumors in different organs of mice (rat).
The development of immunodeficient mice…
The development of immunodeficient mice and the construction method of the humanized mice…
Similar articles
- In Vitro-Transcribed mRNA Chimeric Antigen Receptor T Cell (IVT mRNA CAR T) Therapy in Hematologic and Solid Tumor Management: A Preclinical Update. Soundara Rajan T, Gugliandolo A, Bramanti P, Mazzon E. Soundara Rajan T, et al. Int J Mol Sci. 2020 Sep 6;21(18):6514. doi: 10.3390/ijms21186514. Int J Mol Sci. 2020. PMID: 32899932 Free PMC article. Review.
- Immune Cell Hacking: Challenges and Clinical Approaches to Create Smarter Generations of Chimeric Antigen Receptor T Cells. Elahi R, Khosh E, Tahmasebi S, Esmaeilzadeh A. Elahi R, et al. Front Immunol. 2018 Jul 31;9:1717. doi: 10.3389/fimmu.2018.01717. eCollection 2018. Front Immunol. 2018. PMID: 30108584 Free PMC article. Review.
- Hurdles of CAR-T cell-based cancer immunotherapy directed against solid tumors. Zhang BL, Qin DY, Mo ZM, Li Y, Wei W, Wang YS, Wang W, Wei YQ. Zhang BL, et al. Sci China Life Sci. 2016 Apr;59(4):340-8. doi: 10.1007/s11427-016-5027-4. Epub 2016 Mar 11. Sci China Life Sci. 2016. PMID: 26965525 Review.
- CAR-T cell therapy: current limitations and potential strategies. Sterner RC, Sterner RM. Sterner RC, et al. Blood Cancer J. 2021 Apr 6;11(4):69. doi: 10.1038/s41408-021-00459-7. Blood Cancer J. 2021. PMID: 33824268 Free PMC article. Review.
- [Adoptive therapy with TCR gene-modified T cells for hematological malignancies and solid tumors]. Ikeda H. Ikeda H. Rinsho Ketsueki. 2019;60(6):716-722. doi: 10.11406/rinketsu.60.716. Rinsho Ketsueki. 2019. PMID: 31281165 Review. Japanese.
- Cutaneous Melanoma: An Overview of Physiological and Therapeutic Aspects and Biotechnological Use of Serine Protease Inhibitors. Boleti APA, Jacobowski AC, Monteiro-Alfredo T, Pereira APR, Oliva MLV, Maria DA, Macedo MLR. Boleti APA, et al. Molecules. 2024 Aug 16;29(16):3891. doi: 10.3390/molecules29163891. Molecules. 2024. PMID: 39202970 Free PMC article. Review.
- Mitochondrial-Stem Cell Connection: Providing Additional Explanations for Understanding Cancer. Martinez P, Baghli I, Gourjon G, Seyfried TN. Martinez P, et al. Metabolites. 2024 Apr 17;14(4):229. doi: 10.3390/metabo14040229. Metabolites. 2024. PMID: 38668357 Free PMC article.
- From Chaos to Opportunity: Decoding Cancer Heterogeneity for Enhanced Treatment Strategies. Ottaiano A, Ianniello M, Santorsola M, Ruggiero R, Sirica R, Sabbatino F, Perri F, Cascella M, Di Marzo M, Berretta M, Caraglia M, Nasti G, Savarese G. Ottaiano A, et al. Biology (Basel). 2023 Aug 29;12(9):1183. doi: 10.3390/biology12091183. Biology (Basel). 2023. PMID: 37759584 Free PMC article. Review.
- Preclinical Models of Neuroblastoma-Current Status and Perspectives. Krawczyk E, Kitlińska J. Krawczyk E, et al. Cancers (Basel). 2023 Jun 23;15(13):3314. doi: 10.3390/cancers15133314. Cancers (Basel). 2023. PMID: 37444423 Free PMC article. Review.
- Sung H, Ferlay J, Siegel RL, Laversanne M, Soerjomataram I, Jemal A, et al. . Global cancer statistics 2020: GLOBOCAN estimates of incidence and mortality worldwide for 36 cancers in 185 countries. CA Cancer J Clin (2021) 71(3):209–49. doi: 10.3322/caac.21660 - DOI - PubMed
- Sengupta R, Zaidi SK. AACR cancer progress report 2021: Discovery science driving clinical breakthroughs. Clin Cancer Res (2021) 27(21):5757–9. doi: 10.1158/1078-0432.CCR-21-3367 - DOI - PubMed
- Aldea M, Andre F, Marabelle A, Dogan S, Barlesi F, Soria JC. Overcoming resistance to tumor-targeted and immune-targeted therapies. Cancer Discovery (2021) 11(4):874–99. doi: 10.1158/ 2159-8290.CD -20-1638 - DOI - PubMed
- Robinson NB, Krieger K, Khan FM, Huffman W, Chang M, Naik A, et al. . The current state of animal models in research: A review. Int J Surg (2019) 72:9–13. doi: 10.1016/j.ijsu.2019.10.015 - DOI - PubMed
- Mouse Genome Sequencing Consortium. Waterston RH, Lindblad-Toh K, Birney E, Rogers J, Abril JF, et al. . Initial sequencing and comparative analysis of the mouse genome. Nature (2002) 420(6915):520–62. doi: 10.1038/nature01262 - DOI - PubMed
Publication types
- Search in MeSH
Related information
Grants and funding, linkout - more resources, full text sources.
- Europe PubMed Central
- Frontiers Media SA
- PubMed Central
Molecular Biology Databases
- Mouse Genome Informatics (MGI)
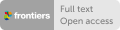
- Citation Manager
NCBI Literature Resources
MeSH PMC Bookshelf Disclaimer
The PubMed wordmark and PubMed logo are registered trademarks of the U.S. Department of Health and Human Services (HHS). Unauthorized use of these marks is strictly prohibited.
REVIEW article
Experimental mouse models for translational human cancer research.
- Department of Thoracic Surgery, The Second Affiliated Hospital, Air Force Medicel University, Xi’an, China
The development and growth of tumors remains an important and ongoing threat to human life around the world. While advanced therapeutic strategies such as immune checkpoint therapy and CAR-T have achieved astonishing progress in the treatment of both solid and hematological malignancies, the malignant initiation and progression of cancer remains a controversial issue, and further research is urgently required. The experimental animal model not only has great advantages in simulating the occurrence, development, and malignant transformation mechanisms of tumors, but also can be used to evaluate the therapeutic effects of a diverse array of clinical interventions, gradually becoming an indispensable method for cancer research. In this paper, we have reviewed recent research progress in relation to mouse and rat models, focusing on spontaneous, induced, transgenic, and transplantable tumor models, to help guide the future study of malignant mechanisms and tumor prevention.
1 Introduction
According to the World Health Organization, there were 19.3 million new cancer cases worldwide in 2020, resulting in nearly 10 million related deaths ( 1 ). Furthermore, with the acceleration in population growth and social aging, the global cancer burden will continue to increase. It is estimated that by 2040, the number of newly diagnosed cancer patients will reach 28.4 million per year, an increase of 47% when compared with 2020 ( 2 ). Recently, new therapeutic strategies, such as immunotherapies and targeted therapies, have significantly improved the survival rates of tumor patients when compared with traditional therapies; however, there are fewer sensitive and beneficial people, and the problem of drug resistance is almost inevitable ( 3 ). Therefore, in-depth exploration of the occurrence and development mechanisms of cancer and the improvement of diagnosis and treatment strategies are of critical global importance, and consequently, this is a major focus of academic research.
Animal tumor models are valuable for experimental analysis as the animal tumors can have similar characteristics to human tumors. We can study the occurrence and development of tumors by observing animal tumor models, and study the effect of genes on tumorigenesis and development by knocking out or knocking in a certain gene, and we can also establish PDX models for drug screening and preclinical trials. Furthermore, this experimental strategy is also advantageous as these experiments are low cost, the animals have short life cycles, and it can help to avoid human experiments. Thus, the use of animal models is extremely valuable for tumor research ( 4 ). At present, based on the causes of tumor formation, we divide the animal model into four categories: spontaneous, induced, transgenic, and transplant. Among them, the spontaneous tumor model, the induced tumor model, and the transgenic model belong to the primary tumor of animals, and the transplantable tumor models include allogeneic transplantation and xenogeneic transplantation; the most used transplantable tumor model is human tumor xenografts in immunodeficient mice. Compared with non-mammals, mammals have a higher degree of similarity to humans, especially mice and rats, and the short reproduction cycle of mice greatly improves the efficiency of these experiments. The mouse genome sequencing project was completed in 2002, and it revealed that 99% of human genes exist in mice; gene homology is as high as 78.5% ( 5 ). Consequently, mice are the most commonly used animals in tumor research. This paper introduces the research progress that has been made with various human tumor models in mice and rats, and analyzes their development processes, advantages and disadvantages, applications, and future development prospects.
2 Animal models for spontaneous tumors
Spontaneous animal tumor models are utilized to investigate tumors that occur naturally in experimental animals without any conscious artificial intervention, and this occurrence type and incidence vary greatly with different species and strains of experimental animal ( Figure 1 ). The advantage of the spontaneous animal tumor model is that the process of tumor formation is similar to that of human tumors, and the long period of tumor occurrence and development can be treated comprehensively with the intervention of many factors, and the role of genetic factors in tumorigenesis can be observed. However, the disadvantage of the spontaneous animal tumor model is that the experimental cycle is long, many experimental animals are required, and the cost is high; in addition, the spontaneous tumors of animals are often heterogeneous and there are individual growth differences, making it difficult to obtain a large number of tumor-bearing animals with uniform growth at the same time.
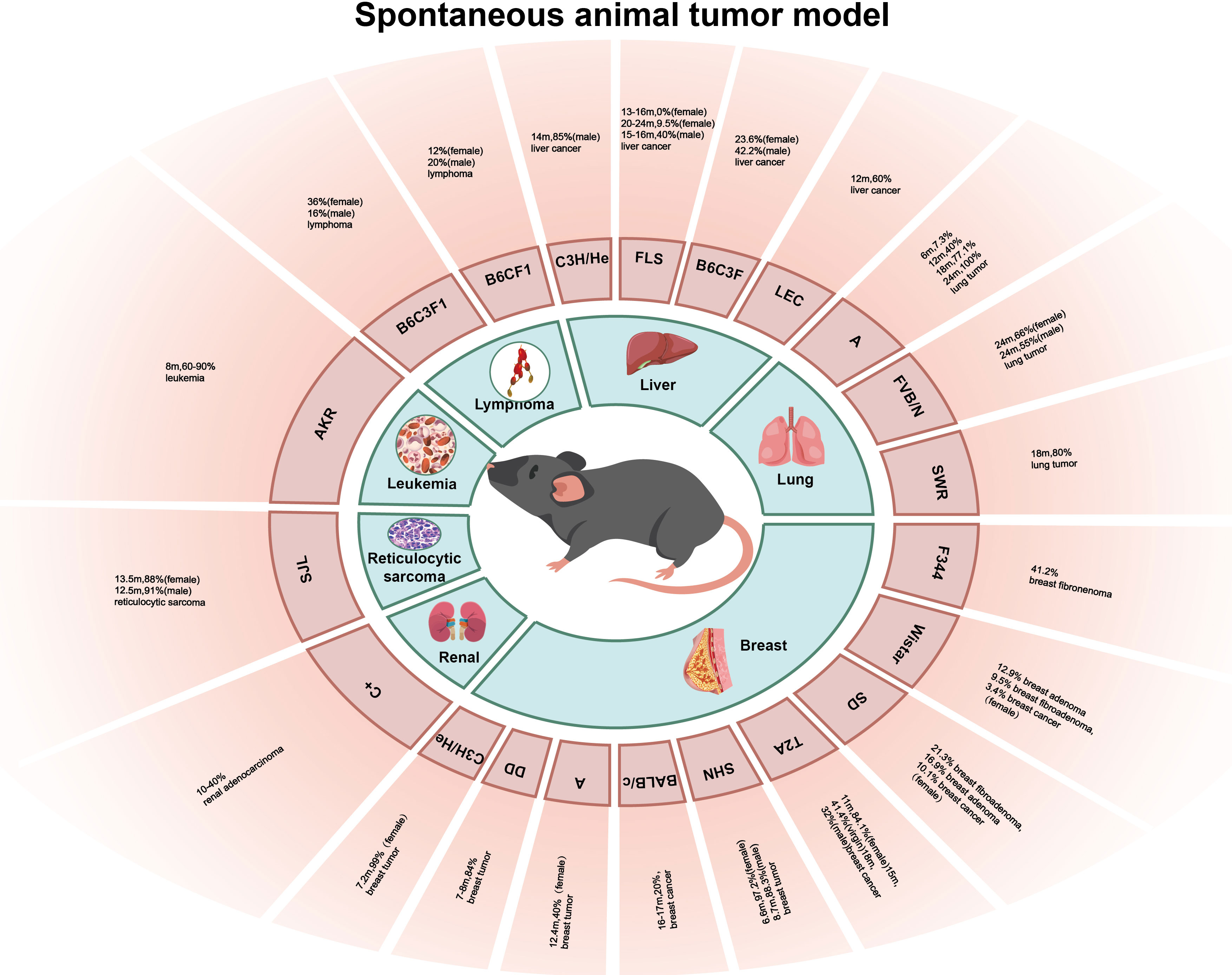
Figure 1 The incidence of various spontaneous tumors in different strains of mice (rat).
2.1 Breast tumors
The incidence and mortality of breast cancer worldwide are increasing and have surpassed lung cancer in 2020 to become the most predominant cancer in the world ( 1 ). Mice have a high incidence of breast cancer ( 6 ). The occurrence of breast tumors in mice is affected by many factors, including factors such as viruses, hormones, heredity, and feed ( 7 ). Mouse mammary tumor virus (MMTV) is the most common cause of breast cancer, which causes breast cancer and lymphoma in mice mainly by insertion mutations and clone amplification in the genome; therefore, mice carrying MMTV have a higher incidence of breast tumors ( 8 ). C3H/He mice were inbred by Strong after crossing Bagg albino female mice with a high incidence of the breast tumor strain DBA male mice in 1920. C3H/He female mice carried MMTV, and the average incidence of spontaneous breast tumors reached 99% at an average of 7.2 months, but the average age of tumorigenesis in the virgin mice was approximately 2 months older than that in postpartum mice ( 9 ). DD mice also carry MMTV, and the incidence of breast tumors is 84% at the age of 7–8 months ( 9 ). BALB/c and other mouse strains do not carry MMTV, and the incidence of breast tumors is low; in 1932, the 26th generation of mice bred by MacDowell from Bagg albino mice through inbred lines was named BALB/c mice, and the incidence of breast cancer in the BALB/c mice was only 20% at 16–17 months ( 9 ), and the incidence of breast tumors in C57BR, C57BL, AKR, and other mice without MMTV was lower ( 10 ). However, some strains of mice without MMTV had a high incidence of breast tumors, such as the A mice, SHN mice, and TA2 mice; A mice were inbred by Strong after inbreeding with the offspring of the local albino mice and Bagg albino mice in 1921, which is a highly spontaneous breast tumor strain, but the A mice do not carry MMTV, and Strain A female mice had an average breast tumor incidence of 40% at the age of 12.4 months ( 9 ). SHN mice were bred by Nagasawa et al. on the basis of the Swiss albino mice, and the incidence of breast tumors in female SHN mice was 97.2% at an average age of 6.6 months, and 88.3% in virgin female SHN mice at 8.7 months ( 10 ). In addition, the inbred strain TA2 mice bred by Sun et al. also had a high incidence of spontaneous breast cancer, and pathological analysis showed that the breast cancer cells of the TA2 mice were triple-negative. The incidence of spontaneous breast cancer reached 84.1% after 11 months in the TA2 mice, 41.4% in the virgin TA2 mice at 15 months, and 32% in the male TA2 mice at 18 months ( 11 ). Wistar, SD, F344, and other rat strains also had a high incidence of breast cancer; Wistar rats were bred by the Wistar Institute of the United States in 1907, and SD rats were bred by Wistar rats in 1925. The incidences of breast fibroadenoma, breast adenoma, and breast cancer in female SD rats were 21.3%, 16.9%, and 10.1%, respectively, while those in female Wistar rats were 12.9%, 9.5%, and 3.4%, respectively ( 12 ). F344 rats were inbred and established by Dunning in subline 344 of the Fischer strain, and the incidence of breast fibroadenoma in the F344 rats was 41.2% ( 13 ).
2.2 Lung tumors
Lung cancer is one of the most common cancers and the leading cause of cancer-related death worldwide, with an estimated 2 million new cases and 1.76 million deaths annually ( 14 ). The histopathological subtypes of lung cancer include non-small cell lung cancer (NSCLC) and small cell lung cancer (SCLC), which can be further divided into adenocarcinoma and squamous cell carcinoma (SCC) ( 15 ). The incidence of spontaneous lung tumors in mice is high, and the pathological types are mainly adenoma and adenocarcinoma, depending on the mouse strain. A mice and SWR mice have a higher incidence of lung tumors, followed by BALB/C mice, CBA mice, and C3H mice, which have a lower incidence of lung tumors, and the lowest incidence of lung tumors was observed in DBA and C57BL/6 mice ( 16 ). A mice are a high spontaneous lung tumor strain, and lung tumors can be detected at 3–4 weeks of age. The incidence of lung tumor was 7.3% (6/83) at 6 months of age, 40.0% (71/178) at 12 months of age, 77.1% (105/136) at 18 months, and almost 100% at 24 months ( 17 ). SWR mice were bred by Lynch et al. in 1926 using Swedish mice for inbreeding. The spontaneous rate of lung tumors in SWR mice over 18 months was 80%, and the incidence of lung tumors in mice whose parents had spontaneous lung tumors was higher than that in mice whose parents had lung tumors alone, while the incidence of lung tumors in mice whose parents had no lung tumors was lower ( 18 ), suggesting a role for genetic factors in tumorigenesis. Although the incidence of lung tumors in SWR mice is high, the long culture period limits the use of this strain in lung tumor research. FVB/N mice were established in the 1970s, and they have a high spontaneous lung tumor rate. The mean incidence of lung tumors in male and female mice at 24 months was 55% and 66%, respectively, and the fertilized egg of FVB/N mice has a large and significant pronucleus and is easy to be microinjected with DNA. Consequently, FVB/N mice have been widely used as animal tumor models ( 19 ).
2.3 Liver tumors
Primary liver cancer is the fourth leading cause of cancer death worldwide, and its incidence is increasing every year. Histologically, it can be divided into hepatocellular carcinoma (HCC) and intrahepatic cholangiocarcinoma ( 20 ). Spontaneous liver tumors in mice often originate from hepatocytes, cholangiocarcinoma is rare, and sarcomas are even rarer. The incidence of liver tumors is different in different strains of mice, but the incidence in male mice is usually higher than that in female mice ( 21 ). Male C3H/He mice are a highly spontaneous liver tumor strain, and Heston et al. found that the incidence of liver cancer in 14-month-old male C3H/He mice could reach 85% ( 9 ). In Japanese FLS mice, inbred mice with non-obese spontaneous fatty liver developed a fatty liver shortly after being fed a normal diet, but were not visibly obese; multiple white protruding nodules appeared in the liver of mice over 12 months of age, and were histologically diagnosed as hepatocytic adenoma and HCC. The incidence of HCC in male mice was 40% at an average of 15–16 months, and that in female Japanese FLS mice was 0% (0/36) at 13–16 months and 9.5% (4/42) at 20–24 months ( 22 ). B6C3F1 mice are the first generation of female C57BL/6 and male C3H hybridizations, and the incidence of liver tumor was 42.2% in male B6C3F1 mice and 23.6% in female B6C3F1 mice ( 13 ). LEC rats are inbred mutant rats established by Joseph A. Long and Herbert M.E. Vans of the University of California, USA. Approximately 40% of LEC rats develop acute posthepatitic death 3–4 months after birth and approximately 60% of rats experience chronic hepatitis and develop HCC a year later ( 23 , 24 ). Some studies have pointed out that the cause of spontaneous hepatitis and HCC in LEC rats is the excessive accumulation of copper in the liver, which produces a large amount of ROS hydroxyl radicals, followed by oxidative stress, which is similar to the development of HCC in humans. Therefore, LEC rats have been widely used in HCC models ( 25 ).
2.4 Other tumors
In 1958, Claude et al. described an animal model of renal adenocarcinoma in C+ mice. The incidence of renal adenocarcinoma in C+ mice was between 10% and 40%, and the incidence of tumors increased with age ( 26 ). The AKR mouse is a new strain established by further inbreeding of AK mice by the Rockefeller Institute in 1936; AKR mice are born with carcinogenic RNA virus, the incidence of leukemia after 8 months is approximately 60%–90%, and that of 18-month-old mice can be as high as 90% ( 27 ). The incidence of reticulocytic sarcoma in the SJL mice was high, and the incidence of reticulocytic sarcoma in 13.5-month-old female and 12.5-month-old male mice was 88% and 91%, respectively ( 28 ). Dragani et al. established hybrid mice (C57BL/6J × C3Hf) F1 (B6C3F1) and (C57BL/6J × BALB/c) F1 (B6CF1); the incidence of lymphoma in male B6C3F1 and B6CF1 mice was 16% and 20%, respectively, and that in female B6C3F1 and B6CF1 mice was 36% and 12%, respectively ( 21 ).
3 Animal models of induced tumor
The induced tumor model refers to the use of exogenous carcinogens to cause changes in the cellular genetic characteristics, resulting in the abnormal growth of active cells and the formation of tumors ( Figure 2 ). Induction methods include physical, chemical, and biological methods, of which the chemical methods are the most extensive and effective for the induction ( Table 1 ), and have the advantages of easy application, short experimental time, and high reproducibility ( 45 ). However, the disadvantage is a high animal mortality rate, and the time, location, and number of lesions are not uniform among individuals.
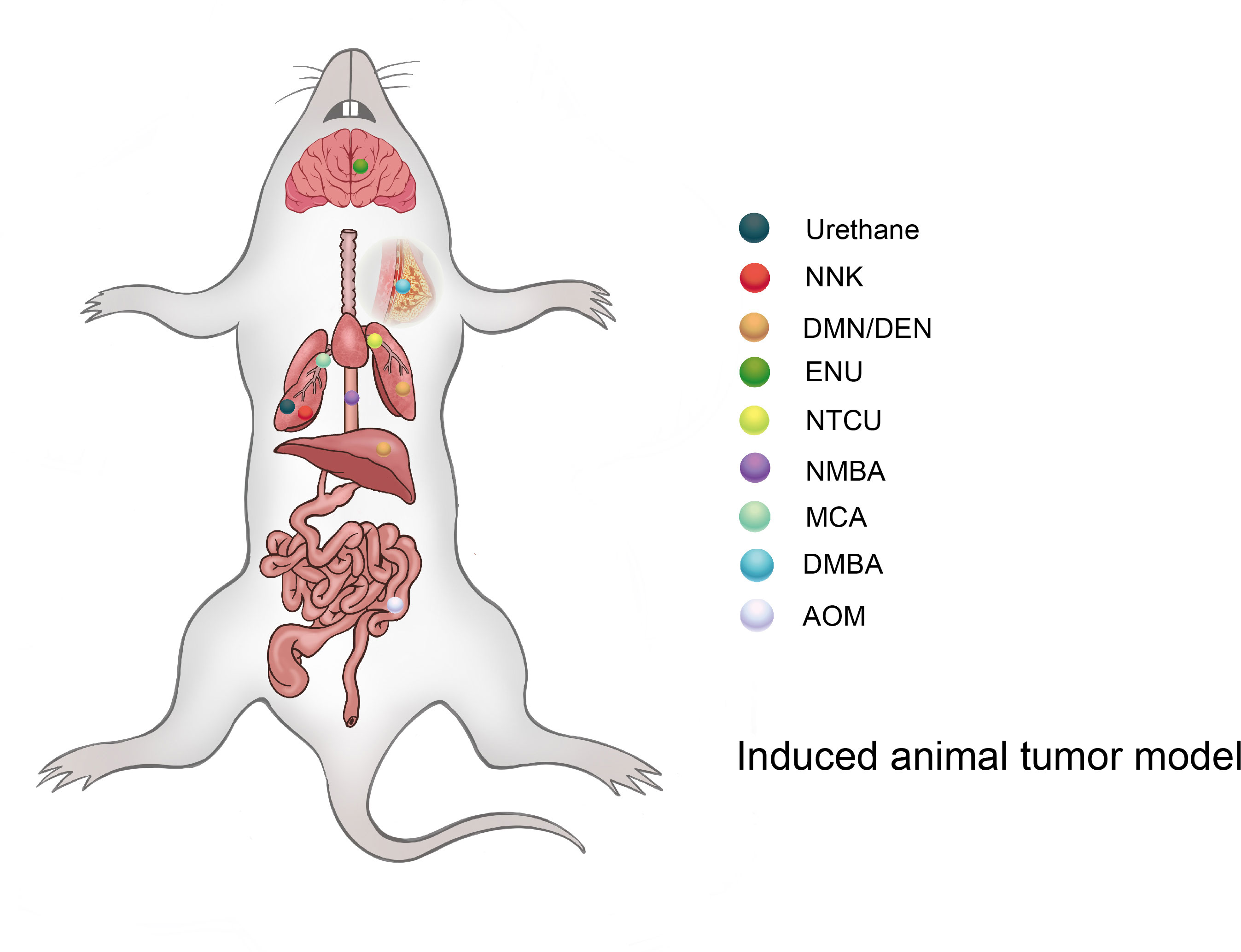
Figure 2 Different types of compounds can induce tumors in different organs of mice (rat).
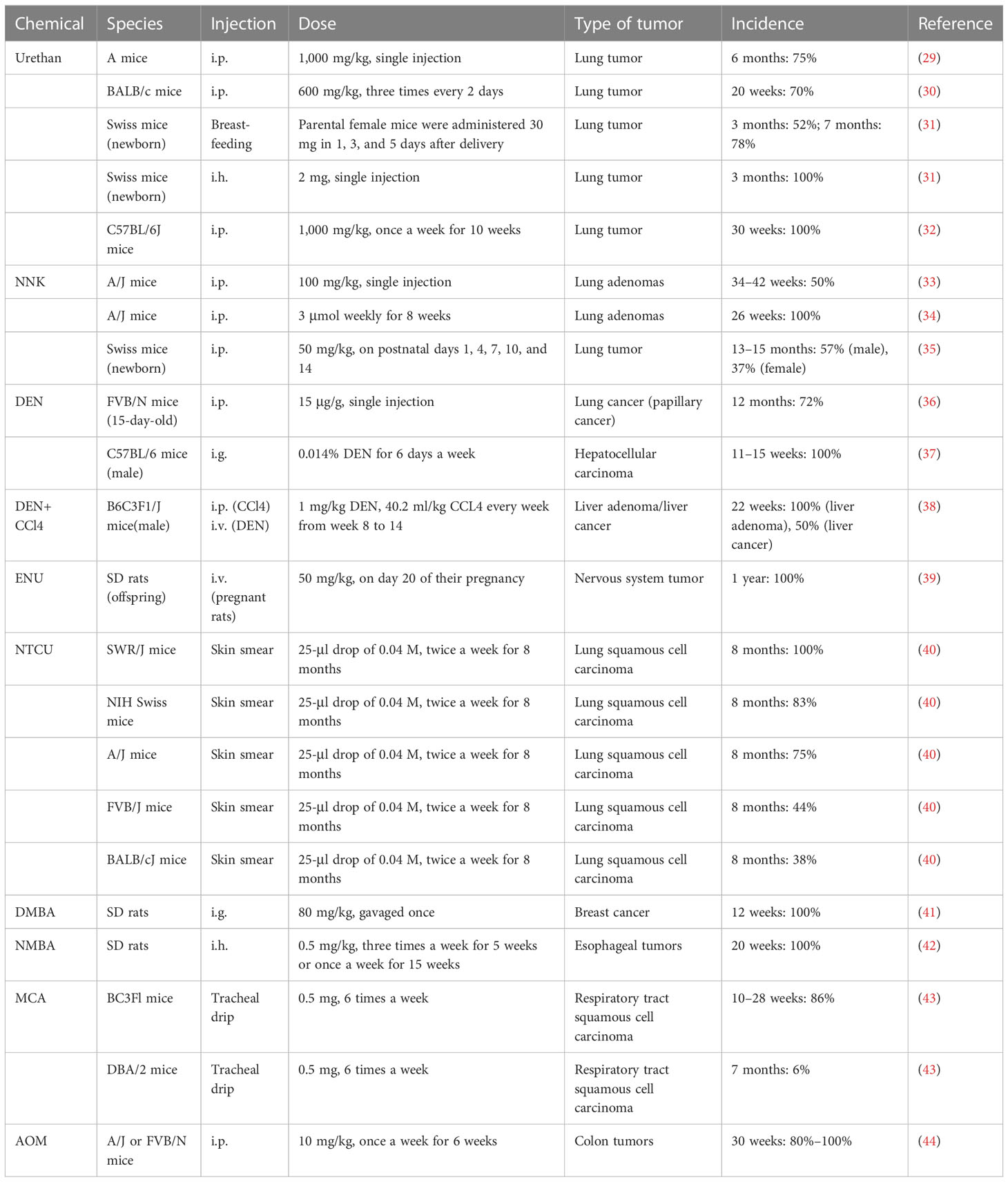
Table 1 Construction method of animal tumor model induced by chemical drugs.
3.1 Urethan
Urethan was originally used as a herbicide and was later found to inhibit cell division and, thus, utilized as a chemotherapeutic drug for leukemia ( 46 ). Urethan is structurally one of the simplest carcinogens; it is soluble in both water and lipids and was the first water-soluble carcinogen to be discovered ( 47 ). The biological effects of urethan depend on the direct inhibition of nucleic acid synthesis, especially of the pyrimidine bases, and an apparent antagonism between urea and pyrimidines also exists ( 47 ). In the 1950s, a study on a model of lung tumors induced by urethan as a carcinogen found that 100% of highly sensitive mice could induce lung tumors ( 48 ). Mice are highly sensitive to urethan, especially newborn mice, and studies have shown that the rate of clearance of the urethan in newborn mice is 1/10 of that in adult mice, and 0.5 mg/g urethan can be metabolized in adult mice within 8 h, while the same dose takes 72 h in newborn mice ( 49 ). Strain A mice have highly spontaneous lung tumor development; while >75% of mice can develop lung tumors at 18 months, 75% of A mice can develop lung tumors at 6 months after a single injection of 1,000 mg/kg urethan ( 29 ). For BALB/c mice, Koohdani et al. injected 600 mg/kg intraperitoneally with urea three times every 2 days and the incidence of lung tumors in the BALB/c mice reached 70% at 20 weeks (7/10) ( 30 ). In 1962, De Benedictis et al. induced lung tumors in Swiss mice, and the parental female mice were administered 30 mg of urea 1, 3, and 5 days after delivery; the results showed that the incidence of lung tumors in the offspring was 52% and 78% at 3 months and 7 months, respectively, and another group of offspring received a single subcutaneous injection of 2 mg in the interscapular area within 24 h after birth, and the incidence of lung tumor was 100% in 3 months ( 31 ). However, the C57BL/6 mice commonly used in genetic engineering have high resistance to lung tumors induced by urethan, and consequently, multiple injections of urethan are required to overcome their genetic resistance and induce lung cancer. C57BL/6J mice were intraperitoneally injected with 1,000 mg/kg once a week for 10 weeks, and the results showed that the incidence of lung tumors in C57BL/6J mice was close to 100% at 30 weeks ( 32 ).
NNK, also known as (methylnitrosamine)-1-(3-pyridine)-1-butanone, is one of the main chemical carcinogens in cigarette smoke ( 50 ), and it can effectively induce lung cancer in mice, rats, and hamsters ( 51 ). The α-hydroxylation of NNK catalyzed by a cytochrome 450 scan easily binds to DNA and forms DNA adducts, causing gene mutations that lead to tumor development ( 33 , 52 ). Belinsky et al. showed that in 6-week-old A/J mice, a single intraperitoneal injection of 100 mg/kg NNK resulted in the development of hyperplasia along the alveolar septum after 14 weeks, and the frequency of lung adenomas in A/J mice at 34–42 weeks resulted in a significant increase to 50% ( 53 ). In another independent study, 7-week-old female A/J mice were given intraperitoneal injections of NNK 3 μmol weekly for 8 weeks, and 100% of the mice developed lung adenomas after 26 weeks ( 34 ). NNK can also induce lung cancer in offspring, as Anderson et al. gave 100 mg/kg NNK doses to female A/J mice on days 14, 16, and 18 of their pregnancies, and it was found that lung tumors occurred in 12 of the 66 offspring and 13 of the 14 female mice ( 54 ). The effects of NNK on young mice were also studied in Swiss mice. Mice were given 50 mg/kg NNK i.p. on postnatal days 1, 4, 7, 10, and 14, and the incidence of lung tumors was 57% in male mice and 37% in female mice at 13–15 months ( 35 ).
3.3 DMN/DEN
Dimethylnitrosamines (DMN) and diethylnitrosamines (DEN) are nitrosamines, which can induce various tumors in mice and rats, but predominantly liver and lung tumors. Nitrosamines are activated by CYP450 enzymes in vivo and converted into a strong alkylating agent, forming adducts in the DNA (active chemicals and cellular macromolecules form stable complexes through covalent bonds), resulting in carcinogenicity ( 55 ). Kohda et al. confirmed the mutagenicity of nitrosamines by treating Chinese hamster V79 cells with nitrosamines ( 56 ). After DEN exposure, A/J mice developed lung adenocarcinoma, 82% of which possessed KRAS mutations ( 57 ). FVB/N mice also have a high incidence of lung cancer, as Zsolt injected 15 μg/g DEN intraperitoneally into 15-day-old FVB/N mice, and the incidence of lung cancer (papillary cancer) was 72% (28/39) after 12 months. Interestingly, there were no mutations in the Kras and EGFR genes ( 36 ). In a study by Chen et al., C57BL/6 male mice were intragastrically administered 0.014% DEN for 6 days a week and given normal drinking water on the 7th day for 15 weeks, and 100% of these mice developed fibrosis (3–6 weeks), cirrhosis (7–10 weeks), and HCC (11–15 weeks) at 3–15 weeks ( 37 ). The incidence of liver tumors caused by the combination of DEN and CCl4 was significantly higher than that of DEN or CCl4 alone. Uehara et al. used 14-day-old B6C3F1/J male mice and administered 1 mg/kg DEN intravenously, and 40.2 ml/kg CCL4 was injected intraperitoneally every week from weeks 8 to 14. The incidence of liver adenoma and liver cancer was 40% and 20% at 17 weeks, and the incidence of liver adenoma and liver cancer was 100% and 50% at 22 weeks ( 38 ). In 1974, Cardesa et al. divided 8-week-old Swiss mice into two groups: subcutaneous injection of DMN or DEN 8 mg/kg; the results showed that the incidence of tumors in mice was 79% (31/39) and 87% (34/39), and the incidence of lung tumors was 67% and 61%, respectively. Lung tumors in the two groups were mainly adenocarcinoma, adenoma, and atypical adenomatoid hyperplasia ( 58 ).
Acetylnitrosourea (ENU) is a strong mutagenic agent that can cause rapid oncogenic genetic mutations in mice ( 59 ). Raju et al. reported that SD rats were administered a single intravenous injection of ENU (50 mg/kg) on day 20 of their pregnancy, and almost 100% of the offspring had central and peripheral nervous system tumors at 1 year of age ( 39 ).
N-nitroso-tris-chloroethylurea (NTCU) is a nitrosoalkylurea compound, which has been shown to induce lung SCC in mice. Wang et al. treated eight different strains of female mice by smearing NTCU on the skin to establish a model. In their study, the back skin of 7-week-old mice was scraped, and they were injected with NTCU 25-μl drop of 0.04 M, twice a week, with a 3-day interval; 8 months later, five strains of the mice had successfully induced lung SCC in situ or lung SCC [the induction rates were as follows: SWR/J, 100% (3/3); NIH Swiss, 83% (10/12); A/J, 75% (6/8); FVB/J, 44% (4/9); and BALB/cJ, 38% (3/8)]. The other strains (AKR/J, 129/svJ, and C57BL/6J) failed to develop the carcinomas, and histological and pathological analysis showed that mouse SCC induced by NTCU had the same pathological process as human SCC, which is “normal-proliferative-metaplastic-abnormal-SCC” ( 40 , 60 ).
Dimethylbenzanthracene (DMBA) is frequently used as a model for polycyclic aromatic hydrocarbon (PAH)-induced mammary tumorigenesis because of its potent carcinogenic and immunosuppressive activities ( 61 ). Female SD rats at the age of 7 weeks were diluted with 80 mg/kg DMBA in 0.5 ml of corn oil and gavaged once, and after 12 weeks, animal models of breast cancer lesions could be established in all rats ( 41 ).
Methyl benzylnitrosamine (NMBA) is also an important carcinogenic compound that is classified as a nitrosamine. NMBA is currently the most effective inducer of rat esophageal tumors, as they can be induced in 15 weeks or less ( 62 ). SD rats were given 0.5 mg/kg NMBA three times a week for 5 weeks or once a week for 15 weeks, and the esophageal tumor incidence was 100% at 20 weeks ( 42 ).
Methylcholanthracene (MCA) is a potent carcinogen that is often used to induce the transformation of cultured cells, and it was found that repeated intratracheal injection of MCA into BC3Fl [(C57BL×C3H)F1] and DBA/2 mice could induce respiratory tract SCC, and the induced SCC had obvious infiltration and metastasis ( 43 ). BC3F1 mice were injected with 0.5 mg of MCA six times a week, and the incidence of respiratory tract SCC was 86% at 10–28 weeks. In contrast, DBA/2 mice that received intratracheal injections of 0.5 mg of MCA four times a week resulted in 6% incidence of SCC of the respiratory tract at 7 months ( 43 ).
Azomethane (AOM) is a chemical reagent that can promote base mismatch and cause cancer through the alkylation of DNA, which is often used in colonic carcinogenicity ( 63 ); 10 mg/kg AOM was injected intraperitoneally into A/J or FVB/N mice once a week for 6 weeks, and resulted in 80%–100% of mice having spontaneous colon tumors within 30 weeks ( 44 ).
3.10 Fattening diet
Nonalcoholic fatty liver disease (NAFLD) is a condition characterized by the excessive accumulation of fat in the liver without chronic alcohol intake; it is estimated that the global prevalence rate is approximately 24% ( 64 ). NAFLD and nonalcoholic steatohepatitis (NASH) are the liver manifestations of metabolic syndrome, and some patients with NAFLD develop into NASH with associated inflammation and fibrosis, which can progress to HCC ( 64 ). Asgharpour et al. successively established a model by following a fattening diet (high fructose-glucose solution and high-fat, high-carbohydrate diet—42% calorie fat and 0.1% cholesterol) in 8- to 12-week-old male mice; 89% of the mice developed HCC at 32–52 weeks, and each mouse had three or more tumor foci ( 65 ).
3.11 Ionizing radiation
Moderate to high doses of radiation are well-established causes of cancer ( 66 ), and ionizing radiation such as x-rays, α-rays, β-rays, and γ-rays can break through genetic material and cause DNA fracture damage and gene mutations ( 67 ). Some studies have shown that irradiated mice may develop a series of malignant tumors, including sarcomas, and single high-dose radiation significantly increases the incidence of tumors when compared with fractionated radiation ( 68 ). Edmondson et al. locally irradiated the right hindlimb of C3Hf/Kam and C57BL/6J mice with a high dose (10–70 Gy) or a fractionated dose (40–80 Gy, 2 Gy per day, five times a week, for 4–8 weeks), and after 800 days, 210 tumors were induced in 788 mice. The observed tumors were primarily sarcoma ( n = 201), and the occurrence frequency of cancer was low ( n = 9). The incidence of tumor after single irradiation was 36.1%, and that of graded irradiation was only16.4% ( 68 ).
4 Transgenic models
In recent years, increasing evidence has shown that gene mutation is an important cause of tumorigenesis, and targeted therapies for driving genes have achieved good results in tumor patients with a specific genetic background, but the problem of drug resistance still restricts the further benefits ( 3 ). Understanding the mechanism of driving mutations in tumorigenesis and development is thus crucial. Due to the high similarity between mouse and human protein coding genes, transgenic mice have been used to study the effects of gene mutations on tumorigenesis and development since the mid-1980s ( 5 ) ( Table 2 ). The advantages of transgenic animal tumor models are that they have great advantages in studying tumorigenesis mechanisms and tumor immune escape. However, the establishment process of transgenic animal models is long, the feeding costs are high, and it is difficult to obtain a large number of experimental animals, which hinders rapid and high-throughput research ( 76 ).
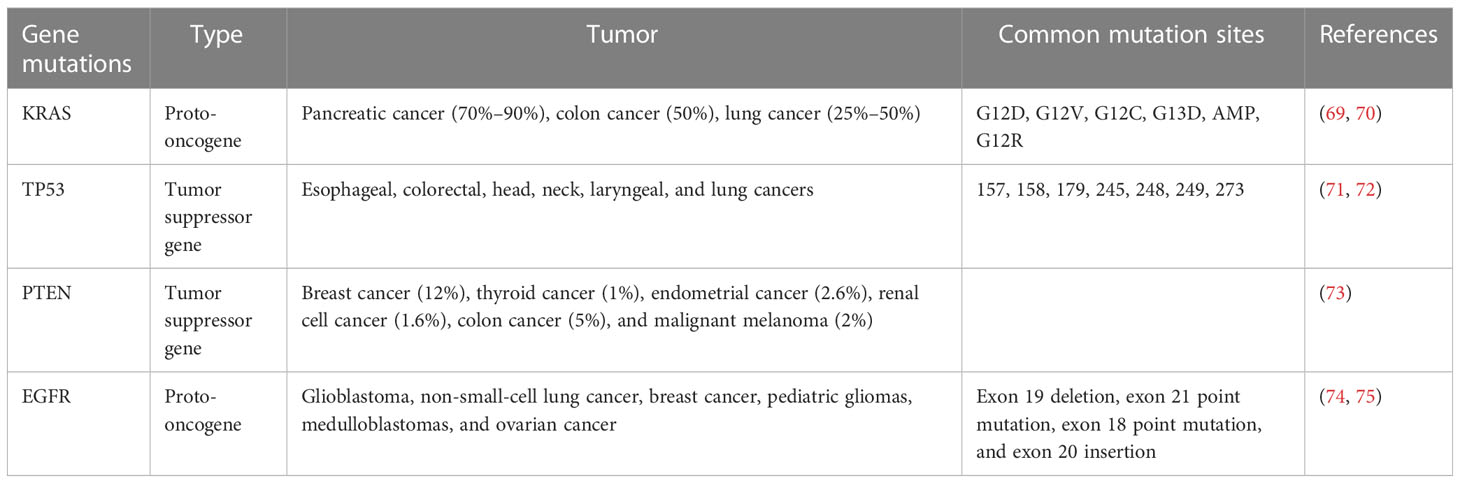
Table 2 The type of tumor caused by gene mutation.
Approximately 30% of human tumors carry ras gene mutations, and the ras gene family includes Kras, Nras, and Hras. Kras is the most commonly mutated gene in the lung, colon, and pancreatic tumors ( 77 ), with a mutation rate of approximately 70%–90% in pancreatic cancer, 50% in colon cancer, 25%–50% in lung cancer ( 69 ), and 15%–25% in lung adenocarcinoma ( 78 , 79 ). Point mutations (including G12D, G12V, G12C, G13D, AMP, and G12R) are the most common Kras mutations ( 70 ). Under normal circumstances, the activation and inactivation of Kras are finely regulated, as wild-type Kras is temporarily activated by tyrosine kinases such as active epidermal growth factor receptor (EGFR). Activated Kras can motivate downstream signaling networks to execute diverse bioactivities, and Kras is then rapidly inactivated ( 80 ). Mutant Kras proteins are uncontrollable, as they can be continuously activated in the absence of an EGFR activation signal, inducing uncontrolled cell proliferation and malignant invasion ( 80 ). Most mutations in lung cancer models are Kras dependent; Kras mutations are found in 90% of spontaneous and chemically induced lung tumors in mice, and genetically engineered Kras mice have been widely used in lung cancer research, which is very similar to the genetic and pathophysiological characteristics of human lung cancer ( 45 , 81 , 82 ). Johnson et al. constructed a new type of mouse with a potential KrasG12D allele (KrasLA), and mice carrying these mutations easily formed a variety of tumor types, predominantly lung tumors, and 100% of mice developed numerous kinds of lung tumors at an early stage ( 68 ). However, KrasLA mice were found to die of respiratory failure caused by lung lesions at a very young age, and the incidence of other tumors is difficult to predict, which restricts the application of this model ( 69 ). Kras gene mutations account for 6% and 18% of diffuse-type and intestinal-type gastric cancers, respectively ( 83 ). Brembeck et al. established Kras transgenic mice under the control of the cytokeratin 19 (K19) promoter; parietal cell decrease and mucous neck cell proliferation were found in 3- to 6-month-old mice ( 84 ). In pancreatic cancer, the Kras mutation occurs in the early stages of tumorigenesis and accounts for approximately 90% of pancreatic ductal adenocarcinomas; it is often combined with other classical mutations (PTEN, etc.) to induce pancreatic cancer, which will be mentioned in the following article ( 85 ).
Somatic mutations in the tumor protein P53 (TP53) gene are the most common changes in human cancers ( 86 ). The incidence of TP53 mutations in ovarian, esophageal, colorectal, head, neck, laryngeal, and lung cancers is 38%–50%, and the mutation rate is approximately 5% in primary leukemia, sarcoma, testicular cancer, malignant melanoma, and cervical cancer, and it is more common in advanced or invasive cancer subtypes ( 71 ). Most TP53 mutations are missense mutations, followed by truncated mutations, intra-frame mutations, and synonymous and uncoded mutations, which are mainly concentrated in known hot spots (the most common sites are 157, 158, 179, 245, 248, 249, and 273) ( 72 ). Normally,TP53 functions by activating or inhibiting the transcription of numerous critical genes in diverse bioactivities, including cell cycle arrest, DNA repair, metabolism, senescence, and apoptosis ( 86 ). Trp53 (TP53 is called Trp53 in mice) knockout mice are common transgenic animal models that formulate spontaneous tumors at the age of 6 months (including breast cancer, sarcoma, brain tumor, and adrenocortical carcinoma) ( 87 ). Compared with homozygous mice, mice with the Trp53 allele heterozygotes had later spontaneous tumors; the most common tumor type in homozygotes was malignant lymphoma, and the main heterozygotes were osteosarcoma and soft tissue sarcoma ( 88 ). In C57BL/6 or 129/Sv mice, the Trp53 deletion preferentially induces sarcoma and lymphoma, and the incidence of breast cancer has gradually increased from 21.4%-46.2% in the fourth generation after backcrossing with BALB/c mice for many generations ( 89 ). It has been reported that the incidence of gastric invasive adenocarcinoma in Trp53 −/− mice is significantly higher than that in WT mice ( 90 ). Ralph et al. established a mouse model of neuroendocrine lung tumors by conditionally inactivating Rb1 and Trp53 in mouse lung epithelial cells, and its morphology and immunophenotype were significantly similar to those of SCLC ( 91 ). However, increasing evidence shows that the TP53 gene still has antitumor effects after the loss of these classical activities ( 92 ). Using a mutant mouse model of p53-3KR (K120R, K161R, and K162R), researchers found that Trp53 can still inhibit cancer initiation, mainly by regulating cell metabolism, despite losing the antitumor effect of inducing cell cycle arrest, apoptosis, and senescence ( 93 ).
Pten (phosphatase and tensin homolog deleted on chromosome ten) is a classical tumor suppressor gene with a mutation rate of approximately 12% in breast cancer, 1% in thyroid cancer, 2.6% in endometrial cancer, 1.6% in renal cell cancer, 5% in colon cancer, and 2% in malignant melanoma ( 73 ). The PTEN deletion was also reported in 15% of poorly differentiated serous ovarian cancers ( 94 ), and Pten mutations were also found in 20% of endometrioid ovarian cancers ( 95 ); approximately 53% of patients with primary bladder cancer showed a decrease or deletion of the Pten protein in the cytoplasm or nucleus of their tumor cells ( 96 ). The tumor inhibitory activity of PTEN depends to a large extent on its phosphatase activity, which regulates the activity of many important cellular pathways, such as PI3K/AKT; thus, it can regulate many cell processes, including proliferation, survival, energy metabolism, cell structure, and movement ( 97 ). The current transgenic model of Pten is widely used in the study of tumorigenesis mechanisms; however, half of the Pten knockout mice died within 1 year after birth, and the rest developed a variety of tumors, including lung, breast, thyroid, endometrial, and prostate cancer, and T-cell lymphoma ( 98 ). AlbCrePten (flox/flox) mice established by Horie et al. using the Cre-loxP system showed hepatocyte specific knockouts of Pten, and AlbCrePten (flox/flox) mice showed huge liver hypertrophy and steatohepatitis, as well as triglyceride accumulation similar to human nonalcoholic steatohepatitis (NASH); at 78 weeks of age, all AlbCrePten (flox/flox) mice had liver adenomas, and 66% of AlbCrePten (flox/flox) mice had HCC ( 99 ). Yanagi et al. specifically knocked out the Pten gene in bronchiolar alveolar epithelial cells of mice under the control of doxycycline to establish SOPten (flox/flox) mice, of which 90% of the SOPten (flox/flox) offspring mice died of hypoxia shortly after birth ( 100 ). Ninety weeks later, all SOPten (flox/flox) mice born showed significant visible lung tumors: 13 tumors were adenocarcinoma and 1 tumor was SCC, as determined by histological examination ( 100 ). Russo et al. found that the Pten deletion increases cell migration, invasion, and upregulation of WNT4, which is a key regulator of Müllerian duct development during embryogenesis ( 101 ). Tsuruta et al. used the Cre-loxP system to specifically knock out the Pten gene in the urine epithelium of mice to obtain FPten (flox/flox) mice; histologically, the urine epithelium cells of the mice showed enlargement of the nucleus and cell volume, and ultimately, approximately 10% of FPten (flox/flox) mice spontaneously developed into pedicled papillary transitional cell carcinoma (TCC) ( 96 ).
Epidermal growth factor receptor (EGFR) is a member of the HER family, which includes HER1 (erbB1, EGFR), HER2 (erbB2, NEU), HER3 (erbB3), and HER4 (erbB4) ( 102 ). Studies have shown that there is high or abnormal expression of EGFR in many solid tumors, which is related to tumor cell proliferation, angiogenesis, invasion, metastasis, and inhibition of apoptosis ( 103 ). In a study using EGFR knockout mice, the types of cells most affected by the EGFR deletion were epithelial cells and glial cells, while the types of cells overexpressing EGFR in the human tumors were epithelial cells and glial cells ( 104 , 105 ). A large number of EGFR mRNA deletions have been observed in a number of neoplasms, first in glioblastoma, but recently in non-small-cell lung cancer, breast cancer, pediatric gliomas, medulloblastomas, and ovarian cancer ( 74 ). There are four main types of EGFR mutations: exon 19 deletion, exon 21 point mutation, exon 18 point mutation, and exon 20 insertion ( 75 ). The most common EGFR mutations are the exon 19 deletion mutations (19DEL) and exon 21 mutations (21L858R), followed by exon 18 G719X, exon 20 S768I, exon 21 L861Q mutation, and T790M mutation in exon 20, which are associated with acquired drug resistance in the first and second generations of EGFR-TKIs ( 75 ). EGFR knockout mice were stunted and died at different developmental stages (implantation, second trimester, or early postpartum), and mainly characterized by epithelial cell defects (including skin, lung, gastrointestinal tract, teeth, and eyelid defects), impaired intestinal proliferation, reduced stem cell area, and mucosal structure disorder ( 105 ). In order to study the role of activated EGFR mutations in lung cancer, Ohashi et al. established transgenic mice carrying EGFR mutations (five nucleotides encoding five amino acids were deleted, which was equivalent to the EGFRdelEN746-A750 mutation found in lung cancer patients) specifically in type II alveolar epithelial cells through its specific SP-C promoter and found that 9 of 47 newborn mice were positive for EGFR gene mutations, 3 mice of the positive type developed lung adenocarcinoma, only 1 mouse carrying lung adenocarcinoma could reproduce, and all of its offspring developed lung tumors after 7 weeks ( 106 ). Ohashi et al. also used EGFR-mutated mouse models to study the evolution of lung adenocarcinoma. Transgenic mice were killed at different time points for pathological examination; atypical adenomatoid hyperplasia (AAH) appeared at 3–4 weeks, diffuse bronchiolo-alveolar carcinoma (BAC) appeared at 4–5 weeks, adenocarcinoma with solid features was observed at 7 weeks, and multiple tumor nodules were observed on the lung surface ( 106 ). Ohashi et al. used a similar method to construct transgenic mice expressing EGFRL858R in type II alveolar epithelial cells; 8 mice had L858R deletion mutations in 27 newborn mice, and 2 mice with the positive mutation developed multifocal adenocarcinomas at 7 weeks. All the offspring of these two mice had BAC at 4–5 weeks and adenocarcinomas at 7 weeks ( 107 ).
4.5 Combined mutations
Tumors are often caused by multiple gene mutations, the most common of which is the activation of oncogenes and inactivation of tumor suppressor genes ( 108 ). Kras and TP53 are common combined gene mutations in human cancer, and KP mice with both Kras and Trp53 mutations are the most classic transgenic model; all KP mice developed primary lung tumors. Six weeks after the lung lesions of mice developed from atypical adenomatous hyperplasia to lung adenomas, the tumors of these mice showed a high degree of nuclear atypia, causing interstitial connective tissue hyperplasia, invasiveness, and metastasis ( 109 ). Kras mutations were found in 95% of pancreatic ductal adenocarcinomas. KPC mice were triple mutants with aKras LSL-G12D , p53 LoxP , and Pdx1-CreER for tamoxifen-inducible pancreatic ductal adenocarcinoma. Pancreatic ductal metaplasia and pancreatic intraepithelial neoplasia occurred in KPC mice at 8–10 weeks, and invasive pancreatic ductal adenocarcinoma occurred at 14–16 weeks, and metastasized to the liver, lung, and peritoneum ( 110 ). Combined mutations of Kras and Pten are also common in human cancers, and the Ptf1a Cre-ERTM , Kras LSL-G12D , and Pten flox , tamoxifen-inducible triple mutant strain (KPP) may be useful as a model for pancreatic adenocarcinoma (PDA)-induced cachexia-a wasting syndrome characterized by the pronounced loss of skeletal and cardiac muscle and adipose tissues ( 111 ). Mutation activation of BRAF is the earliest and most common genetic change in human melanoma; mice specifically expressing BRAF(V600E) showed benign melanocyte proliferation, but did not develop melanoma after 15–20 months, and BRAF(V600E) expression combined with PTEN gene silencing could induce malignant melanoma and metastasis to lymph nodes and lungs ( 112 ). The Rb1 deletion, TP53 deletion, and Myc amplification are all common mutations in SCLC ( 113 ). RPM mice carried three gene mutations: Rb1 fl/fl , Trp53 fl/fl , and Myc LSL/LSL ; 100% of these mice developed SCLC after 6–8 weeks ( 113 ). It is worth noting that these common classical gene mutations are often combined with new gene mutations to study the function of these new genes in carcinogenesis.
5 Transplantable animal tumor models
Human tumor generated mouse models are established by transplanting human tumor cells and/or tissues of research interest into recipient animals (almost always possessing immune function deficiencies) ( Figure 3 ). The advantage is that most types of human tumors can establish transplantable tumor models in immunodeficient animals, and under the same inoculation conditions, the growth rate of animals is the same, the difference in tumor formation rate is small, and the inoculation tumor formation rate is high. The disadvantage is that the recipient host animal needs to be in an immunodeficient state requiring special housing in an aseptic environment, which is expensive to maintain. Furthermore, not all cell types of human tumors can be successfully established in rodent models and the stroma of the human tumor tissue obtained may contain the components of the recipient animals. It is worth noting that the key to developing an optimal transplanted tumor model lies in the immune status of the host (immunodeficiency state) and the composition of the graft (containing important or all components of the tumor).
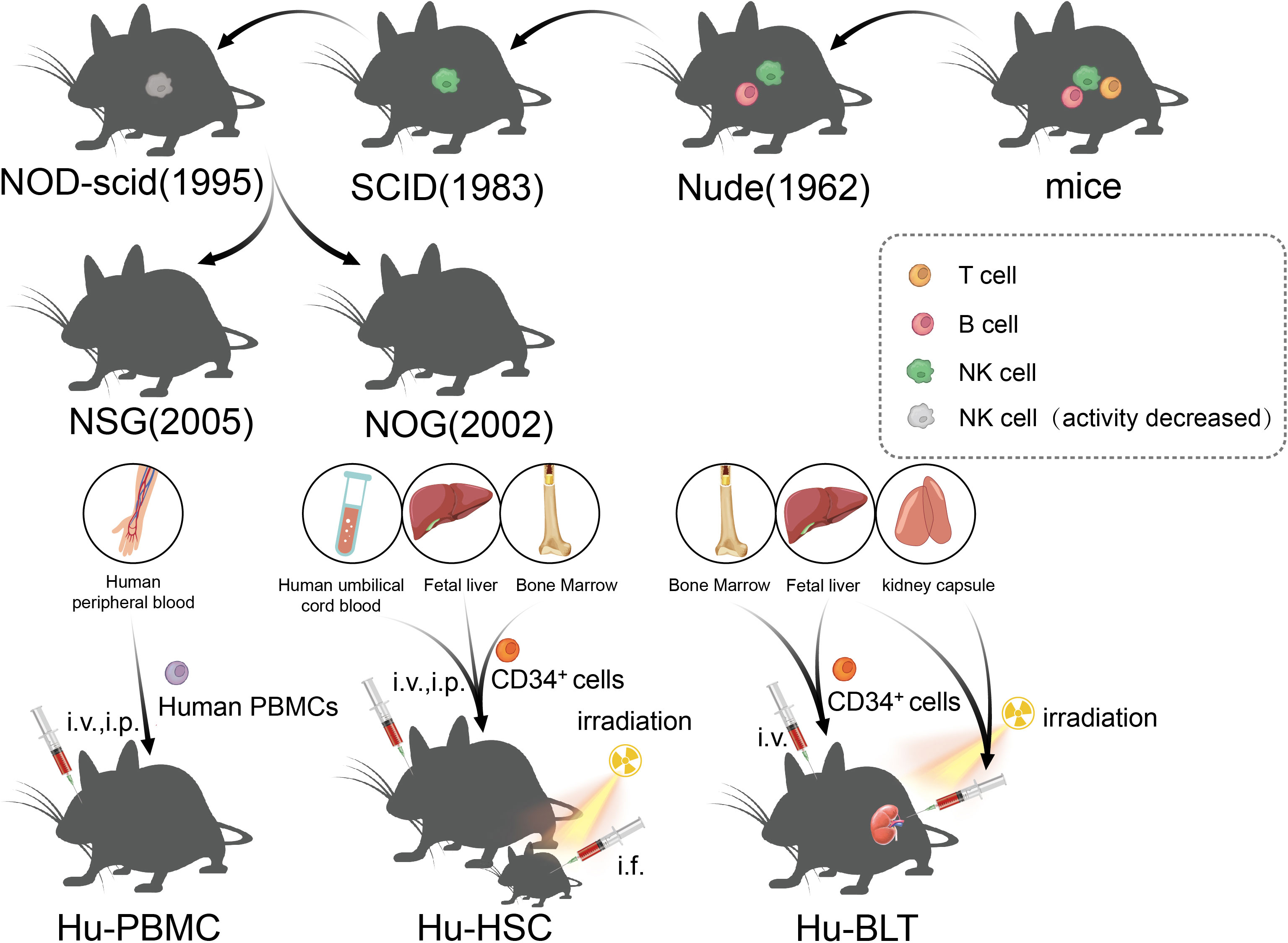
Figure 3 The development of immunodeficient mice and the construction method of the humanized mice model.
5.1 Immunodeficient mice
The immune status of the host has undergone many improvements, which is the key to the development of a successful transplant tumor model. Transplantable tumors are divided into allogeneic transplantation and xenogeneic transplantation, and the most used is the xenotransplantation of human tumors. Due to the existence of immune rejection, wild mice cannot be used for xenotransplantation of human tumor. However, human tumors can be implanted in immunodeficient mice, and the tumors can maintain the histological, immunological, and biological characteristics of original tumors. Nude mice were first identified by Grist in 1962, and they were called nude mice as they are hairless. Flanagan found that nude mice lack thymus and T lymphocytes, and thus, they lacked T cell-mediated immune responses and could be used as recipients for human tumor xenotransplantation ( 114 ). However, because nude mice still have B cells and NK, they are not suitable for use as hosts for lymphoma and leukemia, which limits the extensive application of nude mice in relation to transplanted tumors. Severe combined immunodeficiency (SCID) mice were first reported by Bosma in 1983 ( 115 ). SCID mice are more severely immunodeficient than nude mice, and the mutant genes of the SCID mice were identified in 1996. The maturation defects of B lymphocytes and T lymphocytes in SCID mice were caused by a point mutation in the Prkdc (protein kinase, DNA-activated, catalytic subunit) gene, which causes the affected lymphocytes to die prior to maturation or be cleared by the macrophages, granulocytes, and NK cells in the body ( 116 , 117 ). However, it is usually difficult to establish a successful model for lymphoma and metastatic tumors in SCID mice because of leakage (some SCID mice will restore the function of some T and B lymphocytes with age) ( 118 ). In addition, NK cells and other innate immunoreactive substances in SCID mice were present at high levels, which limits the success rate of transplantation, such as human hematopoietic stem cell (HSC) transplantation ( 119 ). Subsequently, the establishment of NOD/SCID mice ushered in a new breakthrough in transplanted animal tumors, which are a type of spontaneous type 1 diabetic mice, caused by T lymphocytes infiltrating and destroying islets, as well as complement loss and impaired function of NK, macrophages, and dendritic cells ( 120 , 121 ). NOD/SCID mice were established through the hybridization of NOD and SCID mice, and NOD/SCID mice will not develop type 1 diabetes, as they lack an adaptive immune system and the loss of effector T cells; moreover, due to extensive defects in innate and adaptive immunity (low activity of NK cells and loss of T- and B-cell function), NOD/SCID mice have become a stable and excellent animal model for human HSCs and human solid tumor transplantation ( 122 ). At the beginning of the 21st century, scientists introduced IL-2Ry mutations on the basis of NOD/SCID mice, resulting in NSG and NOG mice, which, in addition to being the mice with the highest degree of immunodeficiency at present, could also be used to construct humanized immune system mice to study the antitumor effects of chimeric antigen receptor T-cell immunotherapy (CAR-T) and immune checkpoint inhibitors (ICIs) ( 123 ), and will be discussed in detail later.
Transplant components have also been the focus of transplantable tumor model research in recent years, and in the past, we transplanted tumor cell lines into animals, which are simpler and easier to use, but the defects cannot be ignored: first, this tumor model cannot fully represent the unique characteristics of each cancer patient; furthermore, this model cannot reconstruct the remaining non-tumor cell components in the tumor tissues, which plays an important role in tumorigenesis and development. Consequently, the patient-derived xenotransplantation (PDX) model has been actively generated and applied, and the PDX model can directly implant cancerous tissues from the patient’s tumor into immunodeficient mice, thus preserving both the cell–cell interaction and the tumor microenvironment ( 124 ). The PDX model retains the characteristics of the primary patient tumor, including the gene expression profile and drug response, and offers great advantages for drug screening, biomarker development, and the evaluation of therapeutic effects ( 125 ). The PDX model usually takes approximately 6 months to 2 years to establish, and the success rate varies (10%–90%), depending on the tumor source and disease characteristics ( 125 ). Specifically, invasive, recurrent, and the transplantation rates tend to be higher for highly metastatic tumors ( 126 ). Gastrointestinal tumors (such as colon and pancreatic cancers) have higher transplantation success rates than other cancers, and the transplantation rate in breast cancer is low ( 127 ). The success rates of the PDX model is as follows: colon cancer [63.5% (54/85) in nude mice and 87% (74/85) in NOD/SCID mice] ( 128 , 129 ), pancreatic cancer [61% (42/69) in nude mice and 67% (8/12) in SCID mice] ( 130 , 131 ), and breast cancer [12.5% (25/200) in nude mice and 27% (13/49) in NOD/SCID mice] ( 132 , 133 ).
Animal models also play an important role in drug development and screening. In the past, the average success rate of translating animal research into human clinical trials was approximately 8%, and <5% of antineoplastic drugs were approved to enter the market ( 134 , 135 ). Although the accuracy of the PDX model on drug efficacy and drug resistance was up to 90% ( 136 ), the traditional PDX model takes a long time to construct; owing to this, it cannot quickly reflect the drug sensitivity of patients and cannot meet clinical needs. China Lidi Biological has developed a new rapid PDX drug sensitivity detection technique (OncoVee ® MiniPDX) for screening clinically related programs of cancer, which uses patient-derived tumor cells arranged in hollow fiber capsules after 7 days of subcutaneous culture. The active morphology and pharmacokinetics of the tumor cells in MiniPDX capsules were evaluated systematically, and the morphological and histopathological characteristics of the tumor cells in MiniPDX capsules were consistent with those of the PDX model and primary tumor ( 137 ).
5.3 Humanized mice model
Recently, ICI and CAR-T have enabled new breakthroughs in the treatment of tumors ( 138 , 139 ). Although PDX, which depends on immunodeficient mice, has been widely used in the research of tumor immunity and the development of new therapies, the lack of models for the human immune system and tumor immune microenvironment limits the study of immune mechanisms and transformation of immunotherapy to a great extent. The humanized mouse model is a mouse model that reconstructs the human immune system by implanting human hematopoietic cells, lymphocytes, or tissues into immunodeficient mice, which can effectively reconstruct the human immune system and better simulate the characteristics of human immunity ( 140 ). From the earliest nude mice to the later SCID and NOD/SCID mice, the success rate of human cell implantation has been low either due to the existence of innate immunity or because of high sensitivity to radiation and the limited life cycle. Owing to this, all these models limited the application of immune system-humanized mice in practical research to some extent. In NOG mice, mature T/B cells and NK cells are lacking, complement activity is decreased, and the function of macrophages and dendritic cells is impaired; therefore, NOG mice are an ideal model for human immune cell transplantation ( 141 ). Subsequently, the IL-2 receptor γ mutation was further developed. NSG mice had a complete IL-2 receptor γ chain-invalid allele, similar to NOG mice, with a loss of T/B and NK cells, lack of complement activity, and defects in macrophages and dendritic cells ( 142 ). In addition, other highly immunodeficient mice, such as BRG and BRGS mice, have been established as humanized mouse models that lack T/B cells and NK cells ( 143 ). Although NOG and NSG mice are very useful humanized models, they still lack the ability to reconstruct myeloid and NK cells, and a new generation of super immunodeficiency models HuNOG-EXL and HuNSG-SGM3 have been developed, which can express some growth factors to promote myeloid regeneration ( 142 , 144 ). According to the method of human immune system reconstruction, the humanized mouse model can be divided into three categories: Hu-PBMCs (humanized-peripheral blood mononuclear cells), Hu-HSCs (humanized-hematopoietic stem cells), and Hu-BLTs (humanized-bone marrow, liver, thymus) ( 145 ). The Hu-PBMC model is a simple and economical humanized mouse model in which mature lymphocytes from peripheral blood mononuclear cells (PBMCs) are injected into immunodeficient host mice through intraperitoneal (i.p.) or intravenous (i.v.) injection ( 145 ). This method was first described in CB17-SCID mice in 1988 and has been widely used to study the human immune response in autoimmunity and infectious diseases ( 117 ). However, graft-versus-host disease (GvHD) occurs in the Hu-PBMC model in 2–3 weeks, and the survival time is short ( 146 ). At present, this model is often used to study the activation of human effector T cells and to evaluate immunosuppressive drugs; in the Hu-HSC mouse model, human CD34 + HSCs from human umbilical cord blood, adult bone marrow, or fetal liver were injected into adult mice (intravenous or intrafemoral) or newborn mice (intracardiac or intrahepatic) ( 147 , 148 ), and sublethal irradiation of host mice is needed to eliminate HSC and promote the transfer of human HSC ( 145 ). Fetal liver and umbilical cord blood are the most commonly used sources of human CD34 + HSCs, which are easier to colonize in immunodeficient mice than that in adult HSCs. Although this method can produce a variety of HSCs in adult mice, the number of T cells produced is small and the model does not possess functional immune cells ( 149 ). In newborn mice (less than 4 weeks old), good human cell transplantation can be obtained, and T cells, B cells, macrophages, NK cells, and DCs can be produced ( 150 ). At present, it is believed that the Hu-HSC model can establish the human innate immune system and lymphocytes, with little or no occurrence of GvHD, which can be used for long-term research; however, owing to the species differences between humans and mice, there is a lack of human cytokines in mice, and the development of human stem cells in mice is limited ( 149 ). The method of establishing Hu-BLT is to transplant human fetal liver and thymus tissue into the renal capsule of adult immunodeficient recipient mice after sublethal irradiation, and at the same time, fetal liver or bone marrow-derived CD34 + HSCs from the same individual is injected intravenously (i.v.) into recipient mice ( 151 ). The Hu-BLT model is often used to study adaptive immune responses, such as HIV infection. However, the incidence of GvHD in the Hu-BLT model is higher than that in other CD34 + HSC transplantation models ( 145 ), and complex and precise surgical procedures are required, thus limiting the application of the Hu-BLT model in the research and development of tumor immune drugs.
6 Prospects
The use of animal models to develop human healthcare can be traced back to the 6th century BC ( 152 ). During this time, there have been significant developments in biotechnology and animal models that have contributed to the development of mechanisms of disease and drug discovery. Since the establishment of the earliest spontaneous animal tumor model, we have obtained a great deal of information about tumor generation and progression. However, we have gradually eliminated this method because of its randomness, high cost, and long cycle of tumorigenesis. Then, we used chemical drugs to induce tumors in mice to establish tumor models and study the carcinogenic effect of a certain factor. In fact, induced tumor is the most widely used method for establishing animal tumor models at present, due to the low technical requirements and cost, most of the laboratories can perform this biotechnique. With the development of the transgenic animal model and the PDX model, we have made great progress in understanding specific gene function, building animal models of human diseases, and evaluating the safety and effectiveness of new drugs, and it is hopeful that it will be a bright light for the study of animal tumor models in the future.
However, they still have significant limitations in modeling human cancer, which is mainly reflected in the immune microenvironment and tumor microenvironment, or the more subtle differences caused by species-specific differences. Although we have developed the PDX model and humanized model to reduce these differences, it is urgent that we develop and establish more effective animal tumor models to facilitate more detailed investigation of the tumor development process.
Author contributions
All authors contributed to the article and approved the submitted version.
This study was funded by the National Natural Science Foundation of China (82002421), the Young Talent Program of Tangdu Hospital, Health Research Fund of Shaanxi Province (2021B004), and the Discipline Innovation Development Plan Project of Tangdu Hospital (2021LCYJ005).
Conflict of interest
The authors declare that the research was conducted in the absence of any commercial or financial relationships that could be construed as a potential conflict of interest.
Publisher’s note
All claims expressed in this article are solely those of the authors and do not necessarily represent those of their affiliated organizations, or those of the publisher, the editors and the reviewers. Any product that may be evaluated in this article, or claim that may be made by its manufacturer, is not guaranteed or endorsed by the publisher.
1. Sung H, Ferlay J, Siegel RL, Laversanne M, Soerjomataram I, Jemal A, et al. Global cancer statistics 2020: GLOBOCAN estimates of incidence and mortality worldwide for 36 cancers in 185 countries. CA Cancer J Clin (2021) 71(3):209–49. doi: 10.3322/caac.21660
PubMed Abstract | CrossRef Full Text | Google Scholar
2. Sengupta R, Zaidi SK. AACR cancer progress report 2021: Discovery science driving clinical breakthroughs. Clin Cancer Res (2021) 27(21):5757–9. doi: 10.1158/1078-0432.CCR-21-3367
3. Aldea M, Andre F, Marabelle A, Dogan S, Barlesi F, Soria JC. Overcoming resistance to tumor-targeted and immune-targeted therapies. Cancer Discovery (2021) 11(4):874–99. doi: 10.1158/2159-8290.CD-20-1638
4. Robinson NB, Krieger K, Khan FM, Huffman W, Chang M, Naik A, et al. The current state of animal models in research: A review. Int J Surg (2019) 72:9–13. doi: 10.1016/j.ijsu.2019.10.015
5. Mouse Genome Sequencing Consortium, Waterston RH, Lindblad-Toh K, Birney E, Rogers J, Abril JF, et al. Initial sequencing and comparative analysis of the mouse genome. Nature (2002) 420(6915):520–62. doi: 10.1038/nature01262
6. Zeng L, Li W, Chen CS. Breast cancer animal models and applications. Zool Res (2020) 41(5):477–94. doi: 10.24272/j.issn.2095-8137.2020.095
7. Holland JF, Pogo BG. Mouse mammary tumor virus-like viral infection and human breast cancer. Clin Cancer Res (2004) 10(17):5647–9. doi: 10.1158/1078-0432.CCR-04-1234
8. Parisi F, Freer G, Mazzanti CM, Pistello M, Poli A. Mouse mammary tumor virus (MMTV) and MMTV-like viruses: An in-depth look at a controversial issue. Viruses (2022) 14(5):977. doi: 10.3390/v14050977
9. Heston WE, Vlahakis G. Mammary tumors, plaques, and hyperplastic alveolar nodules in various combinations of mouse inbred strains and the different lines of the mammary tumor virus. Int J Cancer (1971) 7(1):141–8. doi: 10.1002/ijc.2910070116
10. Nagasawa H, Yanai R, Taniguchi H, Tokuzen R, Nakahara W. Two-way selection of a stock of Swiss albino mice for mammary tumorigenesis: establishment of two new strains (SHN and SLN). J Natl Cancer Inst (1976) 57(2):425–30. doi: 10.1093/jnci/57.2.425
11. Sun B, Zhang S, Zhang D, Li Y, Zhao X, Luo Y, et al. Identification of metastasis-related proteins and their clinical relevance to triple-negative human breast cancer. Clin Cancer Res (2008) 14(21):7050–9. doi: 10.1158/1078-0432.CCR-08-0520
12. He YN, Zhang SC, Zhang HM. [Pathologic changes of spontaneous tumors in sprague-dawley and wistar rats]. Zhonghua Bing Li Xue Za Zhi (2017) 46(4):249–54. doi: 10.3760/cma.j.issn.0529-5807.2017.04.007
13. Haseman JK, Hailey JR, Morris RW. Spontaneous neoplasm incidences in Fischer 344 rats and B6C3F1 mice in two-year carcinogenicity studies: a national toxicology program update. Toxicol Pathol (1998) 26(3):428–41. doi: 10.1177/019262339802600318
14. Thai AA, Solomon BJ, Sequist LV, Gainor JF, Heist RS. Lung cancer. Lancet (2021) 398(10299):535–54. doi: 10.1016/S0140-6736(21)00312-3
15. Bade BC, Dela Cruz CS. Lung cancer 2020: Epidemiology, etiology, and prevention. Clin Chest Med (2020) 41(1):1–24. doi: 10.1016/j.ccm.2019.10.001
16. Tuveson DA, Jacks T. Modeling human lung cancer in mice: similarities and shortcomings. Oncogene (1999) 18(38):5318–24. doi: 10.1038/sj.onc.1203107
17. Shimkin MB, Stoner GD. Lung tumors in mice: application to carcinogenesis bioassay. Adv Cancer Res (1975) 21:1–58. doi: 10.1016/S0065-230X(08)60970-7
18. Lynch CJ. Studies on the relation between tumor susceptibility and heredity : Iii. spontaneous tumors of the lung in mice. J Exp Med (1926) 43(3):339–55. doi: 10.1084/jem.43.3.339
19. Mahler JF, Stokes W, Mann PC, Takaoka M, Maronpot RR. Spontaneous lesions in aging FVB/N mice. Toxicol Pathol (1996) 24(6):710–6. doi: 10.1177/019262339602400606
20. Dasgupta P, Henshaw C, Youlden DR, Clark PJ, Aitken JF, Baade PD. Global trends in incidence rates of primary adult liver cancers: A systematic review and meta-analysis. Front Oncol (2020) 10:171. doi: 10.3389/fonc.2020.00171
21. Dragani TA, Sozzi G, Della Porta G. Spontaneous and urethan-induced tumor incidence in B6C3F1 versus B6CF1 mice. Tumori (1984) 70(6):485–90. doi: 10.1177/030089168407000603
22. Soga M, Kishimoto Y, Kawamura Y, Inagaki S, Makino S, Saibara T. Spontaneous development of hepatocellular carcinomas in the FLS mice with hereditary fatty liver. Cancer Lett (2003) 196(1):43–8. doi: 10.1016/S0304-3835(03)00213-1
23. Yoshida MC, Masuda R, Sasaki M, Takeichi N, Kobayashi H, Dempo K, et al. New mutation causing hereditary hepatitis in the laboratory rat. J Hered (1987) 78(6):361–5. doi: 10.1093/oxfordjournals.jhered.a110416
24. Li Y, Togashi Y, Sato S, Emoto T, Kang JH, Takeichi N, et al. Spontaneous hepatic copper accumulation in long-Evans cinnamon rats with hereditary hepatitis. a model of wilson's disease. J Clin Invest (1991) 87(5):1858–61. doi: 10.1172/JCI115208
25. Marquez A, Villa-Trevino S, Gueraud F. The LEC rat: a useful model for studying liver carcinogenesis related to oxidative stress and inflammation. Redox Rep (2007) 12(1):35–9. doi: 10.1179/135100007X162220
26. Claude A. [Morphology and organization of nuclear constituents in a case of renal carcinoma in the mouse]. C R Seances Soc Biol Fil (1961) 252:4186–8.
PubMed Abstract | Google Scholar
27. Kawashima K, Ikeda H, Hartley JW, Stockert E, Rowe WP, Old LJ. Changes in expression of murine leukemia virus antigens and production of xenotropic virus in the late preleukemic period in AKR mice. Proc Natl Acad Sci USA (1976) 73(12):4680–4. doi: 10.1073/pnas.73.12.4680
28. Owens MH, Bonavida B. Immune functions characteristic of SJL/J mice and their association with age and spontaneous reticulum cell sarcoma. Cancer Res (1976) 36(3):1077–83.
29. Cowen PN. Some studies on the action of urethane on mice. Br J Cancer (1947) 1(4):401–5. doi: 10.1038/bjc.1947.39
30. Koohdani F, Sasani F, Mohammad K, Mehdipour P. Comparison of ki-67 antigen expression and K-ras mutation in lung tumours induced by urethane in mice. Singapore Med J (2009) 50(7):729–33.
31. De Benedictis G, Maiorano G, Chiecobianchi L, Fiore-Donati L. Lung carcinogenesis by urethane in newborn, suckling, and adult Swiss mice. Br J Cancer (1962) 16:686–9. doi: 10.1038/bjc.1962.78
32. Sozio F, Schioppa T, Sozzani S, Del Prete A. Urethane-induced lung carcinogenesis. Methods Cell Biol (2021) 163:45–57. doi: 10.1016/bs.mcb.2020.09.005
33. Hecht SS, Carmella SG, Kenney PM, Low SH, Arakawa K, Yu MC. Effects of cruciferous vegetable consumption on urinary metabolites of the tobacco-specific lung carcinogen 4-(methylnitrosamino)-1-(3-pyridyl)-1-butanone in singapore chinese. Cancer Epidemiol Biomarkers Prev (2004) 13(6):997–1004. doi: 10.1158/1055-9965.997.13.6
34. Hecht SS, Isaacs S, Trushin N. Lung tumor induction in A/J mice by the tobacco smoke carcinogens 4-(methylnitrosamino)-1-(3-pyridyl)-1-butanone and benzo[a]pyrene: a potentially useful model for evaluation of chemopreventive agents. Carcinogenesis (1994) 15(12):2721–5. doi: 10.1093/carcin/15.12.2721
35. Anderson LM, Hecht SS, Kovatch RM, Amin S, Hoffmann D, Rice JM. Tumorigenicity of the tobacco-specific carcinogen 4-(methyl-nitrosamino)-1-(3-pyridyl)-1-butanone in infant mice. Cancer Lett (1991) 58(3):177–81. doi: 10.1016/0304-3835(91)90097-2
36. Mervai Z, Egedi K, Kovalszky I, Baghy K. Diethylnitrosamine induces lung adenocarcinoma in FVB/N mouse. BMC Cancer (2018) 18(1):157. doi: 10.1186/s12885-018-4068-4
37. Chen Q, You X, Yang W, Jiang S, Lai J, Zhang H, et al. Survival of endogenous hepatic stem/progenitor cells in liver tissues during liver cirrhosis. Life Sci (2020) 241:117121. doi: 10.1016/j.lfs.2019.117121
38. Uehara T, Ainslie GR, Kutanzi K, Pogribny IP, Muskhelishvili L, Izawa T, et al. Molecular mechanisms of fibrosis-associated promotion of liver carcinogenesis. Toxicol Sci (2013) 132(1):53–63. doi: 10.1093/toxsci/kfs342
39. Raju NR, Yaeger MJ, Okazaki DL, Lovell K, Koestner A. Immunohistochemical characterization of rat central and peripheral nerve tumors induced by ethylnitrosourea. Toxicol Pathol (1990) 18(1Pt 1):18–23. doi: 10.1177/019262339001800103
40. Wang Y, Zhang Z, Yan Y, Lemon WJ, LaRegina M, Morrison C, et al. A chemically induced model for squamous cell carcinoma of the lung in mice: histopathology and strain susceptibility. Cancer Res (2004) 64(5):1647–54. doi: 10.1158/0008-5472.CAN-03-3273
41. Karnam KC, Ellutla M, Bodduluru LN, Kasala ER, Uppulapu SK, Kalyankumarraju M, et al. Preventive effect of berberine against DMBA-induced breast cancer in female sprague dawley rats. BioMed Pharmacother (2017) 92:207–14. doi: 10.1016/j.biopha.2017.05.069
42. Aisner J. Comprehensive textbook of thoracic oncology . Williams & Wilkins (1996).
Google Scholar
43. Nettesheim P, Hammons AS. Induction of squamous cell carcinoma in the respiratory tract of mice. J Natl Cancer Inst (1971) 47(3):697–701.
44. Neufert C, Becker C, Neurath MF. An inducible mouse model of colon carcinogenesis for the analysis of sporadic and inflammation-driven tumor progression. Nat Protoc (2007) 2(8):1998–2004. doi: 10.1038/nprot.2007.279
45. Malyla V, Paudel KR, Shukla SD, Donovan C, Wadhwa R, Pickles S, et al. Recent advances in experimental animal models of lung cancer. Future Med Chem (2020) 12(7):567–70. doi: 10.4155/fmc-2019-0338
46. Field KJ, Lang CM. Hazards of urethane (ethyl carbamate): a review of the literature. Lab Anim (1988) 22(3):255–62. doi: 10.1258/002367788780746331
47. Mirvish SS. The carcinogenic action and metabolism of urethan and n-hydroxyurethan. Adv Cancer Res (1968) 11:1–42. doi: 10.1016/s0065-230x(08)60386-3
48. Malkinson AM, Beer DS. Major effect on susceptibility to urethan-induced pulmonary adenoma by a single gene in BALB/cBy mice. J Natl Cancer Inst (1983) 70(5):931–6.
49. Mirvish SS. The metabolism of n-hydroxyurethane in relation to its carcinogenic action: conversion into urethane and an n-hydroxyurethane glucuronide. Biochim Biophys Acta (1966) 117(1):1–12. doi: 10.1016/0304-4165(66)90146-2
50. Liu Y, Yin T, Feng Y, Cona MM, Huang G, Liu J, et al. Mammalian models of chemically induced primary malignancies exploitable for imaging-based preclinical theragnostic research. Quant Imaging Med Surg (2015) 5(5):708–29. doi: 10.3978/j.issn.2223-4292.2015.06.01
51. Ge GZ, Xu TR, Chen C. Tobacco carcinogen NNK-induced lung cancer animal models and associated carcinogenic mechanisms. Acta Biochim Biophys Sin (Shanghai) (2015) 47(7):477–87. doi: 10.1093/abbs/gmv041
52. Kiyohara C, Yoshimasu K, Takayama K, Nakanishi Y. NQO1, MPO, and the risk of lung cancer: a HuGE review. Genet Med (2005) 7(7):463–78. doi: 10.1097/01.gim.0000177530.55043.c1
53. Belinsky SA, Devereux TR, Foley JF, Maronpot RR, Anderson MW. Role of the alveolar type II cell in the development and progression of pulmonary tumors induced by 4-(methylnitrosamino)-1-(3-pyridyl)-1-butanone in the A/J mouse. Cancer Res (1992) 52(11):3164–73.
54. Anderson LM, Hecht SS, Dixon DE, Dove LF, Kovatch RM, Amin S, et al. Evaluation of the transplacental tumorigenicity of the tobacco-specific carcinogen 4-(methylnitrosamino)-1-(3-pyridyl)-1-butanone in mice. Cancer Res (1989) 49(14):3770–5.
55. Verna L, Whysner J, Williams GM. N-nitrosodiethylamine mechanistic data and risk assessment: bioactivation, DNA-adduct formation, mutagenicity, and tumor initiation. Pharmacol Ther (1996) 71(1-2):57–81. doi: 10.1016/0163-7258(96)00062-9
56. Huang Kohda K, Anjo T, Mochizuki M, Kawazoe Y, Okada M. Mutagenicity of alpha-acetoxy derivatives of n-methyl-N-benzylnitrosamine and N,N-dibenzylnitrosamine in V79 cells: A comparison between methylating and benzylating mutagens. Gan (1982) 73(4):517–21.
57. You M, Wang Y, Lineen AM, Gunning WT, Stoner GD, Anderson MW. Mutagenesis of the K-ras protooncogene in mouse lung tumors induced by n-ethyl-N-nitrosourea or n-nitrosodiethylamine. Carcinogenesis (1992) 13(9):1583–6. doi: 10.1093/carcin/13.9.1583
58. Cardesa A, Pour P, Althoff J, Mohr U. Comparative studies of neoplastic response to a single dose of nitroso compounds. 4. the effect of dimethyl- and diethyl-nitrosamine in Swiss mice. Z Krebsforsch Klin Onkol Cancer Res Clin Oncol (1974) 81(3-4):229–33. doi: 10.1007/BF00305023
59. Salinger AP, Justice MJ. Mouse mutagenesis using n-Ethyl-N-Nitrosourea (ENU). CSH Protoc (2008) 2008:pdb prot4985. doi: 10.1101/pdb.prot4985
60. Wang Y, Zhang Z, Garbow JR, Rowland DJ, Lubet RA, Sit D, et al. Chemoprevention of lung squamous cell carcinoma in mice by a mixture of Chinese herbs. Cancer Prev Res (Phila) (2009) 2(7):634–40. doi: 10.1158/1940-6207.CAPR-09-0052
61. Jung KJ, Wallig MA, Singletary KW. Purple grape juice inhibits 7,12-dimethylbenz[a]anthracene (DMBA)-induced rat mammary tumorigenesis and in vivo DMBA-DNA adduct formation. Cancer Lett (2006) 233(2):279–88. doi: 10.1016/j.canlet.2005.03.020
62. Beer DG, Stoner GD. Clinical models of chemoprevention for the esophagus. Hematol Oncol Clin North Am (1998) 12(5):1055–77. doi: 10.1016/S0889-8588(05)70041-1
63. Papanikolaou A, Shank RC, Delker DA, Povey A, Cooper DP, Rosenberg DW. Initial levels of azoxymethane-induced DNA methyl adducts are not predictive of tumor susceptibility in inbred mice. Toxicol Appl Pharmacol (1998) 150(1):196–203. doi: 10.1006/taap.1998.8393
64. Younossi Z, Anstee QM, Marietti M, Hardy T, Henry L, Eslam M, et al. Global burden of NAFLD and NASH: Trends, predictions, risk factors and prevention. Nat Rev Gastroenterol Hepatol (2018) 15(1):11–20. doi: 10.1038/nrgastro.2017.109
65. Asgharpour A, Cazanave SC, Pacana T, Seneshaw M, Vincent R, Banini BA, et al. A diet-induced animal model of non-alcoholic fatty liver disease and hepatocellular cancer. J Hepatol (2016) 65(3):579–88. doi: 10.1016/j.jhep.2016.05.005
66. Abalo KD, Rage E, Leuraud K, Richardson DB, Le Pointe HD, Laurier D, et al. Early life ionizing radiation exposure and cancer risks: systematic review and meta-analysis. Pediatr Radiol (2021) 51(1):45–56. doi: 10.1007/s00247-020-04803-0
67. Morgan C. Ionizing radiation study. Science (1979) 206(4420):769. doi: 10.1126/science.493980
68. Edmondson EF, Hunter NR, Weil MM, Mason KA. Tumor induction in mice after localized single- or fractionated-dose irradiation: Differences in tumor histotype and genetic susceptibility based on dose scheduling. Int J Radiat Oncol Biol Phys (2015) 92(4):829–36. doi: 10.1016/j.ijrobp.2015.03.002
69. Johnson L, Mercer K, Greenbaum D, Bronson RT, Crowley D, Tuveson DA, et al. Somatic activation of the K-ras oncogene causes early onset lung cancer in mice. Nature (2001) 410(6832):1111–6. doi: 10.1038/35074129
70. Hofmann MH, Gerlach D, Misale S, Petronczki M, Kraut N. Expanding the reach of precision oncology by drugging all KRAS mutants. Cancer Discov (2022) 12(4):924–37. doi: 10.1158/2159-8290.CD-21-1331
71. Petitjean A, Mathe E, Kato S, Ishioka C, Tavtigian SV, Hainaut P, et al. Impact of mutant p53 functional properties on TP53 mutation patterns and tumor phenotype: lessons from recent developments in the IARC TP53 database. Hum Mutat (2007) 28(6):622–9. doi: 10.1002/humu.20495
72. Saleh MM, Scheffler M, Merkelbach-Bruse S, Scheel AH, Ulmer B, Wolf J, et al. Comprehensive analysis of TP53 and KEAP1 mutations and their impact on survival in localized- and advanced-stage NSCLC. J Thorac Oncol (2022) 17(1):76–88. doi: 10.1016/j.jtho.2021.08.764
73. Ngeow J, Eng C. PTEN in hereditary and sporadic cancer. Cold Spring Harb Perspect Med (2020) 10(4):a036087. doi: 10.1101/cshperspect.a036087
74. Voldborg BR, Damstrup L, Spang-Thomsen M, Poulsen HS. Epidermal growth factor receptor (EGFR) and EGFR mutations, function and possible role in clinical trials. Ann Oncol (1997) 8(12):1197–206. doi: 10.1023/A:1008209720526
75. Santos EDS, Nogueira KAB, Fernandes LCC, Martins JRP, Reis AVF, Neto JBV, et al. EGFR targeting for cancer therapy: Pharmacology and immunoconjugates with drugs and nanoparticles. Int J Pharm (2021) 592:120082. doi: 10.1016/j.ijpharm.2020.120082
76. Sharpless NE, Depinho RA. The mighty mouse: genetically engineered mouse models in cancer drug development. Nat Rev Drug Discov (2006) 5(9):741–54. doi: 10.1038/nrd2110
77. Prior IA, Hood FE, Hartley JL. The frequency of ras mutations in cancer. Cancer Res (2020) 80(14):2969–74. doi: 10.1158/0008-5472.CAN-19-3682
78. Riely GJ, Kris MG, Rosenbaum D, Marks J, Li A, Chitale DA, et al. Frequency and distinctive spectrum of KRAS mutations in never smokers with lung adenocarcinoma. Clin Cancer Res (2008) 14(18):5731–4. doi: 10.1158/1078-0432.CCR-08-0646
79. Brose MS, Volpe P, Feldman M, Kumar M, Rishi I, Gerrero R, et al. BRAF and RAS mutations in human lung cancer and melanoma. Cancer Res (2002) 62(23):6997–7000.
80. Reck M, Carbone DP, Garassino M, Barlesi F. Targeting KRAS in non-small-cell lung cancer: recent progress and new approaches. Ann Oncol (2021) 32(9):1101–10. doi: 10.1016/j.annonc.2021.06.001
81. Prior IA, Lewis PD, Mattos C. A comprehensive survey of ras mutations in cancer. Cancer Res (2012) 72(10):2457–67. doi: 10.1158/0008-5472.CAN-11-2612
82. Malkinson AM. Molecular comparison of human and mouse pulmonary adenocarcinomas. Exp Lung Res (1998) 24(4):541–55. doi: 10.3109/01902149809087385
83. Ushijima T, Sasako M. Focus on gastric cancer. Cancer Cell (2004) 5(2):121–5. doi: 10.1016/S1535-6108(04)00033-9
84. Brembeck FH, Moffett J, Wang TC, Rustgi AK. The keratin 19 promoter is potent for cell-specific targeting of genes in transgenic mice. Gastroenterology (2001) 120(7):1720–8. doi: 10.1053/gast.2001.24846
85. Mann KM, Ying H, Juan J, Jenkins NA, Copeland NG. KRAS-related proteins in pancreatic cancer. Pharmacol Ther (2016) 168:29–42. doi: 10.1016/j.pharmthera.2016.09.003
86. Levine AJ. Spontaneous and inherited TP53 genetic alterations. Oncogene (2021) 40(41):5975–83. doi: 10.1038/s41388-021-01991-3
87. Donehower LA, Harvey M, Slagle BL, McArthur MJ, Montgomery CA Jr, Butel JS, et al. Mice deficient for p53 are developmentally normal but susceptible to spontaneous tumours. Nature (1992) 356(6366):215–21. doi: 10.1038/356215a0
88. Harvey M, McArthur MJ, Montgomery CA Jr, Butel JS, Bradley A, Donehower LA. Spontaneous and carcinogen-induced tumorigenesis in p53-deficient mice. Nat Genet (1993) 5(3):225–9. doi: 10.1038/ng1193-225
89. Machida Y, Sudo Y, Uchiya N, Imai T. Increased susceptibility to mammary carcinogenesis and an opposite trend in endometrium in Trp53 heterozygous knockout female mice by backcrossing the BALB/c strain onto the background C3H strain. J Toxicol Pathol (2019) 32(3):197–203. doi: 10.1293/tox.2018-0057
90. Ohgaki H, Fukuda M, Tohma Y, Huang H, Stoica G, Tatematsu M, et al. Effect of intragastric application of n-methylnitrosourea in p53 knockout mice. Mol Carcinog (2000) 28(2):97–101. doi: 10.1002/1098-2744(200006)28:2<97::AID-MC5>3.0.CO;2-O
91. Meuwissen R, Linn SC, Linnoila RI, Zevenhoven J, Mooi WJ, Berns A. Induction of small cell lung cancer by somatic inactivation of both Trp53 and Rb1 in a conditional mouse model. Cancer Cell (2003) 4(3):181–9. doi: 10.1016/S1535-6108(03)00220-4
92. Liu Y, Gu W. The complexity of p53-mediated metabolic regulation in tumor suppression. Semin Cancer Biol (2021) 85:4–32. doi: 10.1016/j.semcancer.2021.03.010
93. Li T, Kon N, Jiang L, Tan M, Ludwig T, Zhao Y, et al. Tumor suppression in the absence of p53-mediated cell-cycle arrest, apoptosis, and senescence. Cell (2012) 149(6):1269–83. doi: 10.1016/j.cell.2012.04.026
94. Hanrahan AJ, Schultz N, Westfal ML, Sakr RA, Giri DD, Scarperi S, et al. Genomic complexity and AKT dependence in serous ovarian cancer. Cancer Discovery (2012) 2(1):56–67. doi: 10.1158/2159-8290.CD-11-0170
95. Cho KR. Ovarian cancer update: lessons from morphology, molecules, and mice. Arch Pathol Lab Med (2009) 133(11):1775–81. doi: 10.5858/133.11.1775
96. Tsuruta H, Kishimoto H, Sasaki T, Horie Y, Natsui M, Shibata Y, et al. Hyperplasia and carcinomas in pten-deficient mice and reduced PTEN protein in human bladder cancer patients. Cancer Res (2006) 66(17):8389–96. doi: 10.1158/0008-5472.CAN-05-4627
97. Worby CA, Dixon JE. Pten. Annu Rev Biochem (2014) 83:641–69. doi: 10.1146/annurev-biochem-082411-113907
98. Di Cristofano A, Kotsi P, Peng YF, Cordon-Cardo C, Elkon KB, Pandolfi PP. Impaired fas response and autoimmunity in pten+/- mice. Science (1999) 285(5436):2122–5. doi: 10.1126/science.285.5436.2122
99. Horie Y, Suzuki A, Kataoka E, Sasaki T, Hamada K, Sasaki J, et al. Hepatocyte-specific pten deficiency results in steatohepatitis and hepatocellular carcinomas. J Clin Invest (2004) 113(12):1774–83. doi: 10.1172/JCI20513
100. Yanagi S, Kishimoto H, Kawahara K, Sasaki T, Sasaki M, Nishio M, et al. Pten controls lung morphogenesis, bronchioalveolar stem cells, and onset of lung adenocarcinomas in mice. J Clin Invest (2007) 117(10):2929–40. doi: 10.1172/JCI31854
101. Russo A, Czarnecki AA, Dean M, Modi DA, Lantvit DD, Hardy L, et al. PTEN loss in the fallopian tube induces hyperplasia and ovarian tumor formation. Oncogene (2018) 37(15):1976–90. doi: 10.1038/s41388-017-0097-8
102. Schlessinger J. Ligand-induced, receptor-mediated dimerization and activation of EGF receptor. Cell (2002) 110(6):669–72. doi: 10.1016/S0092-8674(02)00966-2
103. Kumar R, George B, Campbell MR, Verma N, Paul AM, Melo-Alvim C, et al. HER family in cancer progression: From discovery to 2020 and beyond. Adv Cancer Res (2020) 147:109–60. doi: 10.1016/bs.acr.2020.04.001
104. Sibilia M, Kroismayr R, Lichtenberger BM, Natarajan A, Hecking M, Holcmann M. The epidermal growth factor receptor: from development to tumorigenesis. Differentiation (2007) 75(9):770–87. doi: 10.1111/j.1432-0436.2007.00238.x
105. Sibilia M, Wagner EF. Strain-dependent epithelial defects in mice lacking the EGF receptor. Science (1995) 269(5221):234–8. doi: 10.1126/science.7618085
106. Ohashi K, Rai K, Fujiwara Y, Osawa M, Hirano S, Takata K, et al. Induction of lung adenocarcinoma in transgenic mice expressing activated EGFR driven by the SP-c promoter. Cancer Sci (2008) 99(9):1747–53. doi: 10.1111/j.1349-7006.2008.00875.x
107. Ohashi K, Takigawa N, Osawa M, Ichihara E, Takeda H, Kubo T, et al. Chemopreventive effects of gefitinib on nonsmoking-related lung tumorigenesis in activating epidermal growth factor receptor transgenic mice. Cancer Res (2009) 69(17):7088–95. doi: 10.1158/0008-5472.CAN-08-4205
108. Iranzo J, Martincorena I, Koonin EV. Cancer-mutation network and the number and specificity of driver mutations. Proc Natl Acad Sci USA (2018) 115(26):E6010–9. doi: 10.1073/pnas.1803155115
109. Jackson EL, Olive KP, Tuveson DA, Bronson R, Crowley D, Brown M, et al. The differential effects of mutant p53 alleles on advanced murine lung cancer. Cancer Res (2005) 65(22):10280–8. doi: 10.1158/0008-5472.CAN-05-2193
110. Maddipati R, Stanger BZ. Pancreatic cancer metastases harbor evidence of polyclonality. Cancer Discovery (2015) 5(10):1086–97. doi: 10.1158/2159-8290.CD-15-0120
111. Talbert EE, Cuitiño MC, Ladner KJ, Rajasekerea PV, Siebert M, Shakya R, et al. Modeling human cancer-induced cachexia. Cell Rep (2019) 28(6):1612–1622.e4. doi: 10.1016/j.celrep.2019.07.016
112. Dankort D, Curley DP, Cartlidge RA, Nelson B, Karnezis AN, Damsky WE Jr, et al. Braf(V600E) cooperates with pten loss to induce metastatic melanoma. Nat Genet (2009) 41(5):544–52. doi: 10.1038/ng.356
113. Mollaoglu G, Guthrie MR, Böhm S, Brägelmann J, Can I, Ballieu PM, et al. MYC drives progression of small cell lung cancer to a variant neuroendocrine subtype with vulnerability to aurora kinase inhibition. Cancer Cell (2017) 31(2):270–85. doi: 10.1016/j.ccell.2016.12.005
114. Flanagan SP. 'Nude', a new hairless gene with pleiotropic effects in the mouse. Genet Res (1966) 8(3):295–309. doi: 10.1017/s0016672300010168
115. Bosma GC, Custer RP, Bosma MJ. A severe combined immunodeficiency mutation in the mouse. Nature (1983) 301(5900):527–30. doi: 10.1038/301527a0
116. McCune JM, Namikawa R, Kaneshima H, Shultz LD, Lieberman M, Weissman IL. The SCID-hu mouse: murine model for the analysis of human hematolymphoid differentiation and function. Science (1988) 241(4873):1632–9. doi: 10.1126/science.241.4873.1632
117. Mosier DE, Gulizia RJ, Baird SM, Wilson DB. Transfer of a functional human immune system to mice with severe combined immunodeficiency. Nature (1988) 335(6187):256–9. doi: 10.1038/335256a0
118. Shigematsu T, Wolf RE, Granger DN. T-Lymphocytes modulate the microvascular and inflammatory responses to intestinal ischemia-reperfusion. Microcirculation (2002) 9(2):99–109. doi: 10.1080/mic.9.2.99.109
119. Miao M, Masengere H, Yu G, Shan F. Reevaluation of NOD/SCID mice as NK cell-deficient models. BioMed Res Int 2021 (2021) p:8851986. doi: 10.1155/2021/8851986
CrossRef Full Text | Google Scholar
120. Makino S, Kunimoto K, Muraoka Y, Mizushima Y, Katagiri K, Tochino Y. Breeding of a non-obese, diabetic strain of mice. Jikken Dobutsu (1980) 29(1):1–13. doi: 10.1538/expanim1978.29.1_1
121. Kikutani H, Makino S. The murine autoimmune diabetes model: NOD and related strains. Adv Immunol (1992) 51:285–322. doi: 10.1016/S0065-2776(08)60490-3
122. Shultz LD, Schweitzer PA, Christianson SW, Gott B, Schweitzer IB, Tennent B, et al. Multiple defects in innate and adaptive immunologic function in NOD/LtSz-scid mice. J Immunol (1995) 154(1):180–91. doi: 10.4049/jimmunol.154.1.180
123. Shultz LD, Ishikawa F, Greiner DL. Humanized mice in translational biomedical research. Nat Rev Immunol (2007) 7(2):118–30. doi: 10.1038/nri2017
124. Jung J, Seol HS, Chang S. The generation and application of patient-derived xenograft model for cancer research. Cancer Res Treat (2018) 50(1):1–10. doi: 10.4143/crt.2017.307
125. Okada S, Vaeteewoottacharn K, Kariya R. Application of highly immunocompromised mice for the establishment of patient-derived xenograft (PDX) models. Cells (2019) 8(8):889. doi: 10.3390/cells8080889
126. Collins AT, Lang SH. A systematic review of the validity of patient derived xenograft (PDX) models: the implications for translational research and personalised medicine. PeerJ (2018) 6:e5981. doi: 10.7717/peerj.5981
127. Murayama T, Gotoh N. Patient-derived xenograft models of breast cancer and their application. Cells (2019) 8(6):621. doi: 10.3390/cells8060621
128. Julien S, Merino-Trigo A, Lacroix L, Pocard M, Goéré D, Mariani P, et al. Characterization of a large panel of patient-derived tumor xenografts representing the clinical heterogeneity of human colorectal cancer. Clin Cancer Res (2012) 18(19):5314–28. doi: 10.1158/1078-0432.CCR-12-0372
129. Bertotti A, Migliardi G, Galimi F, Sassi F, Torti D, Isella C, et al. A molecularly annotated platform of patient-derived xenografts ("xenopatients") identifies HER2 as an effective therapeutic target in cetuximab-resistant colorectal cancer. Cancer Discov (2011) 1(6):508–23. doi: 10.1158/2159-8290.CD-11-0109
130. Mattie M, Christensen A, Chang MS, Yeh W, Said S, Shostak Y, et al. Molecular characterization of patient-derived human pancreatic tumor xenograft models for preclinical and translational development of cancer therapeutics. Neoplasia (2013) 15(10):1138–50. doi: 10.1593/neo.13922
131. Garrido-Laguna I, Uson M, Rajeshkumar NV, Tan AC, de Oliveira E, Karikari C, et al. Tumor engraftment in nude mice and enrichment in stroma- related gene pathways predict poor survival and resistance to gemcitabine in patients with pancreatic cancer. Clin Cancer Res (2011) 17(17):5793–800. doi: 10.1158/1078-0432.CCR-11-0341
132. Marangoni E, Vincent-Salomon A, Auger N, Degeorges A, Assayag F, de Cremoux P, et al. A new model of patient tumor-derived breast cancer xenografts for preclinical assays. Clin Cancer Res (2007) 13(13):3989–98. doi: 10.1158/1078-0432.CCR-07-0078
133. DeRose YS, Wang G, Lin YC, Bernard PS, Buys SS, Ebbert MT, et al. Tumor grafts derived from women with breastcancer authentically reflect tumor pathology, growth, metastasis and disease outcomes. Nat Med (2011) 17(11):1514–20. doi: 10.1038/nm.2454
134. Mak IW, Evaniew N, Ghert M. Lost in translation: animal models and clinical trials in cancer treatment. Am J Transl Res (2014) 6(2):114–8.
135. DiMasi JA, Reichert JM, Feldman L, Malins A. Clinical approval success rates for investigational cancer drugs. Clin Pharmacol Ther (2013) 94(3):329–35. doi: 10.1038/clpt.2013.117
136. Tentler JJ, Tan AC, Weekes CD, Jimeno A, Leong S, Pitts TM, et al. Patient-derived tumour xenografts as models for oncology drug development. Nat Rev Clin Oncol (2012) 9(6):338–50. doi: 10.1038/nrclinonc.2012.61
137. Zhang F, Wang W, Long Y, Liu H, Cheng J, Guo L, et al. Characterization of drug responses of mini patient-derived xenografts in mice for predicting cancer patient clinical therapeutic response. Cancer Commun (Lond) (2018) 38(1):60. doi: 10.1186/s40880-018-0329-5
138. Portuguese AJ, Tykodi SS, Blosser CD, Gooley TA, Thompson JA, Hall ET. Immune checkpoint inhibitor use in solid organ transplant recipients: A systematic review. J Natl Compr Canc Netw (2022) 20(4):406–416.e11. doi: 10.6004/jnccn.2022.7009
139. Huang R, Li X, He Y, Zhu W, Gao L, Liu Y, et al. Recent advances in CAR-T cell engineering. J Hematol Oncol (2020) 13(1):86. doi: 10.1186/s13045-020-00910-5
140. Shultz LD, Brehm MA, Garcia-Martinez JV, Greiner DL. Humanized mice for immune system investigation: progress, promise and challenges. Nat Rev Immunol (2012) 12(11):786–98. doi: 10.1038/nri3311
141. Ito M, Hiramatsu H, Kobayashi K, Suzue K, Kawahata M, Hioki K, et al. NOD/SCID/gamma(c)(null) mouse: an excellent recipient mouse model for engraftment of human cells. Blood (2002) 100(9):3175–82. doi: 10.1182/blood-2001-12-0207
142. Shultz LD, Lyons BL, Burzenski LM, Gott B, Chen X, Chaleff S, et al. Human lymphoid and myeloid cell development in NOD/LtSz-scid IL2R gamma null mice engrafted with mobilized human hemopoietic stem cells. J Immunol (2005) 174(10):6477–89. doi: 10.4049/jimmunol.174.10.6477
143. Legrand N, Huntington ND, Nagasawa M, Bakker AQ, Schotte R, Strick-Marchand H, et al. Functional CD47/signal regulatory protein alpha (SIRP(alpha)) interaction is required for optimal human T- and natural killer- (NK) cell homeostasis in vivo. Proc Natl Acad Sci USA (2011) 108(32):13224–9. doi: 10.1073/pnas.1101398108
144. Jangalwe S, Shultz LD, Mathew A, Brehm MA. Improved b cell development in humanized NOD-scid IL2Rgamma(null) mice transgenically expressing human stem cell factor, granulocyte-macrophage colony-stimulating factor and interleukin-3. Immun Inflamm Dis (2016) 4(4):427–40. doi: 10.1002/iid3.124
145. De La Rochere P, Guil-Luna S, Decaudin D, Azar G, Sidhu SS, Piaggio E. Humanized mice for the study of immuno-oncology. Trends Immunol (2018) 39(9):748–63. doi: 10.1016/j.it.2018.07.001
146. King MA, Covassin L, Brehm MA, Racki W, Pearson T, Leif J, et al. Human peripheral blood leucocyte non-obese diabetic-severe combined immunodeficiency interleukin-2 receptor gamma chain gene mouse model of xenogeneic graft-versus-host-like disease and the role of host major histocompatibility complex. Clin Exp Immunol (2009) 157(1):104–18. doi: 10.1111/j.1365-2249.2009.03933.x
147. Traggiai E, Chicha L, Mazzucchelli L, Bronz L, Piffaretti JC, Lanzavecchia A, et al. Development of a human adaptive immune system in cord blood cell-transplanted mice. Science (2004) 304(5667):104–7. doi: 10.1126/science.1093933
148. Holyoake TL, Nicolini FE, Eaves CJ. Functional differences between transplantable human hematopoietic stem cells from fetal liver, cord blood, and adult marrow. Exp Hematol (1999) 27(9):1418–27. doi: 10.1016/S0301-472X(99)00078-8
149. Watanabe Y, Takahashi T, Okajima A, Shiokawa M, Ishii N, Katano I, et al. The analysis of the functions of human b and T cells in humanized NOD/shi-scid/gammac(null) (NOG) mice (hu-HSC NOG mice). Int Immunol (2009) 21(7):843–58. doi: 10.1093/intimm/dxp050
150. Brehm MA, Bortell R, Diiorio P, Leif J, Laning J, Cuthbert A, et al. Human immune system development and rejection of human islet allografts in spontaneously diabetic NOD-Rag1null IL2rgammanull Ins2Akita mice. Diabetes (2010) 59(9):2265–70. doi: 10.2337/db10-0323
151. Xia J, Hu Z, Yoshihara S, Li Y, Jin CH, Tan S, et al. Modeling human leukemia immunotherapy in humanized mice. EBioMedicine (2016) 10:101–8. doi: 10.1016/j.ebiom.2016.06.028
152. Ericsson AC, Crim MJ, Franklin CL. A brief history of animal modeling. Mo Med (2013) 110(3):201–5.
Keywords: tumor, immune microenvironment, animal model, spontaneity, induced, transgene, PDX, immunodeficiency
Citation: Zhou Y, Xia J, Xu S, She T, Zhang Y, Sun Y, Wen M, Jiang T, Xiong Y and Lei J (2023) Experimental mouse models for translational human cancer research. Front. Immunol. 14:1095388. doi: 10.3389/fimmu.2023.1095388
Received: 11 November 2022; Accepted: 20 February 2023; Published: 10 March 2023.
Reviewed by:
Copyright © 2023 Zhou, Xia, Xu, She, Zhang, Sun, Wen, Jiang, Xiong and Lei. This is an open-access article distributed under the terms of the Creative Commons Attribution License (CC BY) . The use, distribution or reproduction in other forums is permitted, provided the original author(s) and the copyright owner(s) are credited and that the original publication in this journal is cited, in accordance with accepted academic practice. No use, distribution or reproduction is permitted which does not comply with these terms.
*Correspondence: Jie Lei, [email protected] ; Yanlu Xiong, [email protected] ; Tao Jiang, [email protected]
† These authors have contributed equally to this work
Disclaimer: All claims expressed in this article are solely those of the authors and do not necessarily represent those of their affiliated organizations, or those of the publisher, the editors and the reviewers. Any product that may be evaluated in this article or claim that may be made by its manufacturer is not guaranteed or endorsed by the publisher.

Request an appointment Clinical Trials Give Now
Ginny L. Clements Breast Cancer Research Institute
Experimental Mouse
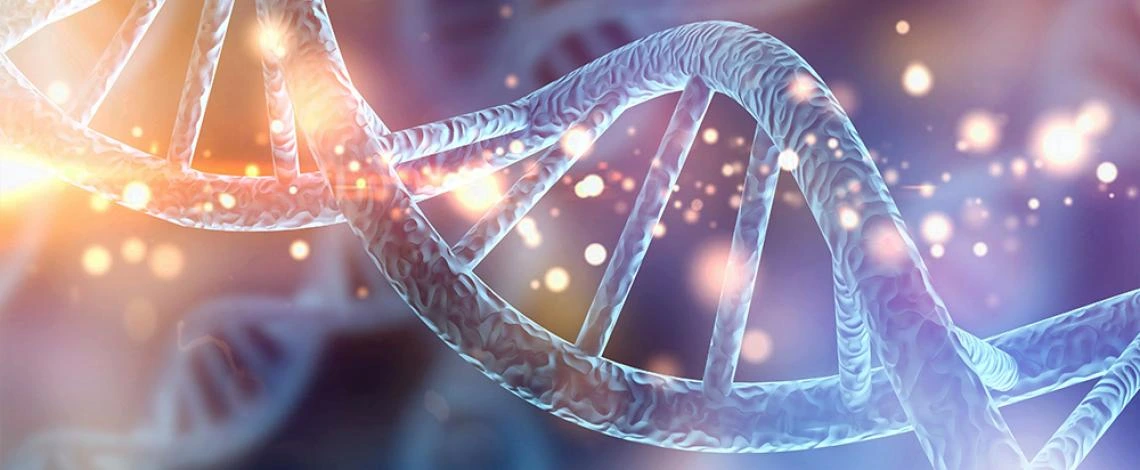
The Experimental Mouse Shared Resource (EMSR) provides expertise to the University of Arizona Cancer Center and outside investigators since 1993. The EMSR offers a continuum of services including initial consultation and in vivo experimental design, Genetically Engineered Mouse (GEM) production, mouse experimentation and data analysis. The EMSR enables the investigator to generate and use mouse models of cancer to their fullest potential, utilizing the service’s extensive knowledge and expertise to develop cancer therapies that are efficient, cost effective, and clinically relevant.
The EMSR is a full-service facility, which performs pre-clinical experiments in a wide variety of in vivo and in vitro cancer models. The team provides technical and scientific expertise in modeling of cancer disease and assists in developing efficient, cost effective, and clinically relevant drug therapies.
To build a project, get a quote, or order a service within iLab:
- Step 1: Select “ About Our Core ” to learn more about the Genetically Engineered Mouse Model Unit. Select " About Our Core " to learn more about the Rodent Experimentation Unit here.
- Step 2: Login or sign up for an iLab account at the University of Arizona. For login help, email [email protected] .
- Step 3: Once the login step is completed, obtaining prices, services and scheduling is available within the iLab system.
Please remember to acknowledge the Cancer Center Support Grant (P30 CA023074 ) when publishing manuscripts or abstracts that utilized the services of the University of Arizona Cancer Center’s Shared Resources and/or were derived from CCSG pilot funds. Suggested language: "Research reported in this [publication/press release] was supported by the National Cancer Institute of the National Institutes of Health under award number P30 CA023074 .
Services provided by the GEM PRODUCTION UNIT:
- vector design and construction for both transgenic and gene-targeted mice
- gene-targeting in ES cells
- screening for targeted ES cells
- CRISPR/Cas9-based genome editing
- pronuclear and cytoplasmic zygote injections and blastocyst injection
- screening for founder, germline chimeric and genome edited mice, and breeding for speed congenics
- consultation on GEM colony management
- sperm cryopreservation, IVF, and embryo rederivation
Prices: Refer to iLab .
Services provided by the RODENT EXPERIMENTATION UNIT:
- in vivo studies
- animal techniques (drug testing, rodent acute toxicity and pharmacokinetics)
- patient-derived xenograft (PDX models)
- surgeries (orthotopic injections, ovariectomy, window chambers, etc.)
- mouse colonies breeding and maintenance
- cell culture and Document Mycoplasma Sample SOP 256a.pdf
- xenograft and GEM models tissue bank
- IACUC protocol support
- administrative support
- media program
- X-ray irradiation of in vitro and in vivo experiments
- Document cell line repository.pdf
Prices: Refer to iLab .
If your desired service is not listed, please contact us by email or call 520-626-7401 .
Timeline for GEM models
The University of Arizona Cancer Center Genetically Engineered Mouse Production Unit Co-Director
T eodora Georgieva, PhD
The Rodent Experimentation Unit Co-Director
N oel Warfel, PhD
Lab Manager
Gillian D. Paine-Murrieta [email protected] 520-626-7401 office 520-626-2346 lab
External Websites
GEMM CORE University of Arizona Genetics Core
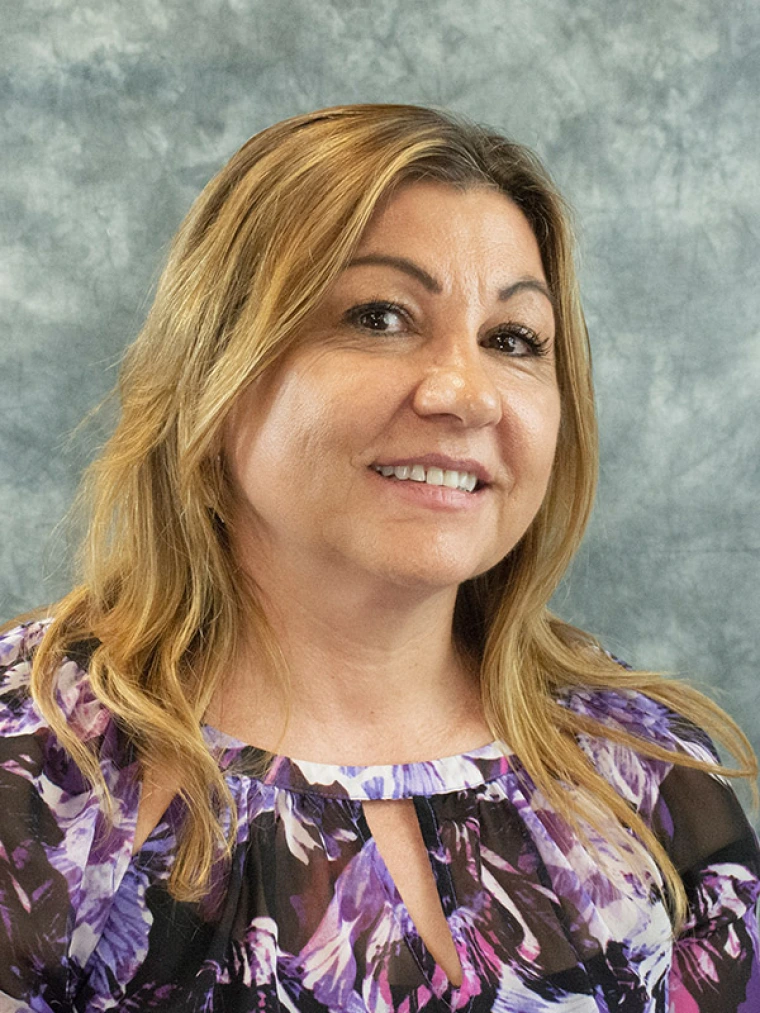
Teodora Georgieva, PhD
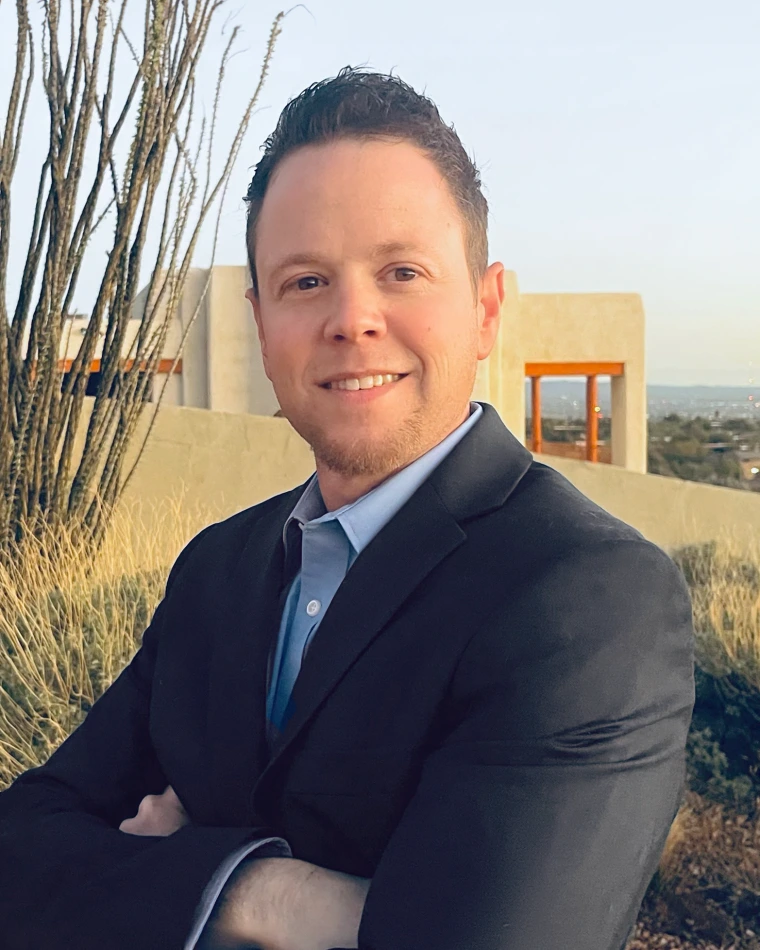
Noel A Warfel, PhD
Login to your account
If you don't remember your password, you can reset it by entering your email address and clicking the Reset Password button. You will then receive an email that contains a secure link for resetting your password
If the address matches a valid account an email will be sent to __email__ with instructions for resetting your password
Property | Value |
---|---|
Status | |
Version | |
Ad File | |
Disable Ads Flag | |
Environment | |
Moat Init | |
Moat Ready | |
Contextual Ready | |
Contextual URL | |
Contextual Initial Segments | |
Contextual Used Segments | |
AdUnit | |
SubAdUnit | |
Custom Targeting | |
Ad Events | |
Invalid Ad Sizes |
Access provided by
Interleukin-34-orchestrated tumor-associated macrophage reprogramming is required for tumor immune escape driven by p53 inactivation

- Standard PDF
- Extended PDF
- Download PDF Download PDF

Graphical abstract

- tumor immune escape
- tumor-associated macrophage
- liver cancer
- tumor immune microenvironment
- cancer stem cell
- foam-like macrophage
- immune checkpoint inhibitor
Introduction
Il-34 is required for tumorigenesis driven by p53 inactivation.

Loss of p53 function directly triggers the secretion of IL-34

IL-34-induced pro-tumoral foam-like macrophages accumulate near the CSCs with p53 inactivation

IL-34 promotes fatty acid uptake via CD36 and induces pro-tumoral polarization of macrophages through FAO metabolic reprogramming

IL-34 orchestrates CD36-mediated metabolic reprogramming to drive pro-tumoral polarization of TAMs in Trp53 null tumors

IL-34-orchestrated TAMs suppress T cell-mediated antitumor immunity to promote tumor immune escape

Blockade of IL-34 signaling serves as a potential immunotherapy for cancer with TP53 mutations

Limitations of the study
Resource availability, lead contact, materials availability, data and code availability, acknowledgments, author contributions, declaration of interests, star★methods, key resources table.
REAGENT or RESOURCE | SOURCE | IDENTIFIER | |
---|---|---|---|
Mouse anti-p53 antibody | CST | Cat# 2524; RRID: | |
InVivoMAb anti-PD-1 antibody | BioXcell | Cat# BE0273; RRID: | |
InVivoMAb anti-IL-34 antibody | R&D | Cat# MAB5195; RRID: | |
InVivoMAb anti-CD36 antibody | Cayman | Cat# 10009893; RRID: | |
InVivoMAb anti-CD8 antibody | BioXcell | Cat# BE0061; RRID: | |
FITC anti-mouse Ly6C | BD | Cat# 553104; RRID: | |
FITC anti-mouse PD-1 | eBioscience | Cat# 11-9985; RRID: | |
PE anti-mouse CD206 | Biolegend | Cat# 141706; RRID: | |
PE-Cy7 anti-mouse CD45 | Biolegend | Cat# 103114; RRID: | |
APC anti-mouse CD3 | BD | Cat# 553066; RRID: | |
APC anti-mouse CD36 | Biolegend | Cat# 102612; RRID: | |
BV421 anti-mouse CD11b | Biolegend | Cat# 101236; RRID: | |
BV605 anti-mouse F4/80 | BD | Cat# 743281; RRID: | |
BV421 anti-mouse TNF-α | Biolegend | Cat# 506328; RRID: | |
BV605 anti-mouse IFN-γ | Biolegend | Cat# 505839; RRID: | |
BV510 anti-mouse CRIg (Vsig4) | BD | Cat# 749505; RRID: | |
BV786 anti-mouse Tim4 | BD | Cat# 742778; RRID: | |
Rabbit Anti-Mouse p21 | Abcam | Cat# ab188224; RRID: | |
Rabbit Anti-IL-34 | Abcam | Cat# ab75723; RRID: | |
Rabbit Anti-GAPDH | Sangon | Cat# D110016; RRID: | |
Rabbit Anti-CD36 | Abcam | Cat# ab252922; RRID: | |
Rabbit Anti-β Actin | proteintech | Cat# 10230-1-AP; RRID: | |
Rabbit Anti-Mouse F4/80 | CST | Cat# 70076; RRID: | |
Rabbit Anti-Mouse PCNA | CST | Cat# 13110; RRID: | |
Rabbit Anti-Mouse EpCAM | Abcam | Cat# ab221552; RRID: | |
Rabbit Anti-Mouse CK19 | Abcam | Cat# ab52625; RRID: | |
Rabbit Anti-Mouse CCR2 | Abcam | Cat# ab273050; RRID: | |
Rabbit Anti-Mouse CD163 | Abcam | Cat# ab182422; RRID: | |
Rabbit Anti-CD36 | Proteintech | Cat# 18836-1-AP; RRID: | |
Rabbit Anti-Mouse CD3ε | CST | Cat# 78588; RRID: | |
Rabbit Anti-Mouse CD47 | Abcam | Cat# ab175388; RRID: | |
HRP-conjugated Goat Anti-Rabbit IgG | Sangon | Cat# D110058; RRID: | |
HRP-conjugated Goat Anti-Mouse IgG | Sangon | Cat# D110087; RRID: | |
Purified Anti-Mouse CD28 | BD | Cat# 553294; RRID: AB 394763 | |
Purified Anti-Mouse CD3 | BD | Cat# 553057; RRID: AB 394590 | |
Human liver cancer samples | First Affiliated Hospital of Anhui Medical University | N/A | |
CCl4 | Aladdin | CAS: 56-23-5 | |
DMEM | VivaCell | Cat# C3103-0500 | |
Fetal Bovine serum (FBS) | Gibco | Cat# 10091148 | |
Penicillin-Streptomycin Liquid | Solarbio | Cat# P1400 | |
ITS liquid media supplement | Sigma | Cat# I3146 | |
L-glutamine | Gibco | Cat# A2916801 | |
DMEM/F12 | VivaCell | Cat# C3132-0500 | |
Dexamethasone | Sigma | Cat# D4902 | |
SYBR Green | Accurate Biology | Cat# AG11741 | |
Foxp3/transcription factor staining buffer | eBioscience | Cat# 00-5523-00 | |
RIPA buffer | Thermo Fisher | Cat# 89901 | |
Phosphatase inhibitor cocktail | APEXBIO | Cat# K1012 | |
PMSF | Beyotime | Cat# ST506 | |
Super Signal West Pico Kit | Thermo Fisher | Cat# 34094 | |
RPMI-1640 | VivaCell | Cat# C3001-0500 | |
M-CSF | Peprotech | Cat# 315-02 | |
IL-34 | R&D | Cat# 5195-ML-010/CF | |
Sulfosuccinimidyl oleate sodium | MCE | Cat# HY-112847A; CAS:1212012-37-7 | |
Etomoxir | Topscience | Cat# T4535L; CAS:124083-20-1 | |
T0070907 | Selleck | Cat# S2871; CAS: 313516-66-4 | |
MitoTracker Deep Red | Thermo Fisher | Cat# M22426 | |
BODIPY FL C12 | Thermo Fisher | Cat# D3822 | |
BODIPY 493/503 | Thermo Fisher | Cat# D3922 | |
DNA transfection reagent | Neofect | Cat# TF201201 | |
TRIzols reagent | Thermo Fisher | Cat# 15596018CN | |
Clodronate liposomes | FormuMax | Cat# F70101C-NC-2 | |
CFSE | Thermo Fisher | Cat# C34570 | |
Mouse tumor dissociation kit | Miltenyi Biotec | Cat# 130-096-730 | |
GentleMACS Dissociator | Miltenyi | Cat# 130-093-235 | |
Mycoplasma Detection Kit | TransGen | Cat# FM311-01 | |
RNA extraction kit | Omega Bio-Tek | Cat# R6934 | |
Reverse transcription kit | Accurate Biology | Cat# AG11705 | |
Chip kit | Absin | Cat# abs50034 | |
Biotin-Streptomycin Complex System | Zhongshan Golden Bridge | Cat# SP-9001 | |
Four-color multiplex fluorescent immunohistochemical staining kit | Absin | Cat# abs50028 | |
IL-34 ELISA kit | R&D | Cat# M3400 | |
TGF-β ELISA kit | Dakewe | Cat# 1217102 | |
IL-10 ELISA kit | Dakewe | Cat# 1211002 | |
M-CSF ELISA kit | Multisciences | Cat# EK2144 | |
IFN-γ ELISA kit | Multisciences | Cat# EK280 | |
BCA Kit | Thermo Fisher | Cat# 23227 | |
Dual luciferase assay kit | Promega | Cat# E1910 | |
Mouse T Cell Isolation Kit | STEM CELL | Cat# 19851 | |
Anti-F4/80 MicroBeads | Miltenyi | Cat# 130-110-443 | |
RNA-seq data | This paper | ||
Chip-seq data | This paper | ||
Spatial transcriptomic data | This paper | ||
snRNA-seq data | This paper | ||
scRNA-seq data of human liver cancer | Xue et al. | ||
Human: 293T cell | Shanghai Cell Bank | Cat# SCSP-502 | |
Mouse: (CT) cell | This paper | N/A | |
Mouse: (PT) cell | This paper | N/A | |
Human: HepG2 cell | Shanghai Cell Bank | Cat# SCSP-510 | |
Mouse: AML12 cell | Procell | Cat# CL-0602 | |
Mouse: RAW264.7 cell | Procell | Cat# CL-0190 | |
Mouse: C57BL/6 | Shanghai SLAC Laboratory Animal Company | N/A | |
Mouse: (C57BL/6) | Shanghai Model Organisms Center | NM-KO-190501 | |
Mouse: -Cre | Shanghai Model Organisms Center | NM-KI-215037 | |
Mouse: -Flox | Shanghai Model Organisms Center | NM-CKO-200086 | |
See for genotyping of mice, -Cre mice and -Flox mice | – | N/A | |
See for sgRNA target site sequences and shRNA sequences | – | N/A | |
See for PCR primer pairs used for qPCR, ChIP assay, DNA pull-down, and sanger sequencing | – | N/A | |
pX330 vector | Addgene | Cat# 42230 | |
LentiCRISPRv2GFP vector | Addgene | Cat# 82416 | |
PB-EF1α-MCS-IRES-GFP | System Biosciences | Cat# PB530A-2 | |
Transposase vector | System Biosciences | Cat# PB220PA-1 | |
PCDH-CMV-MCS-EF1α-copGFP | System Biosciences | Cat# CD511B-1 | |
PLKO.1-GFP vector | Addgene | Cat# 30323 | |
ps.pAX2 vector | Addgene | Cat# 12260; RRID: Addgene_12260 | |
pMD.2G vector | Addgene | Cat# 12259; RRID: Addgene_12259 | |
pGL3.basic vector | Promega | Cat# E1751 | |
pRL-SV40 vectors | Promega | Cat# E2231 | |
FlowJo v10.1 | Tree Star | ||
GraphPad Prism v8.0 | GraphPad Software | ||
CaseViewer | 3DHISTECH | ||
LightCycler 96 SW 1.1 | Roche | ||
ZEN 2 | Carl Zeiss Microscopy | ||
Biorender | Biorender | ||
Cell Ranger | 10X Genomics | ||
Seurat | Butler et al. | ||
inferCNV | Github | ||
cytoTRACE | Gulati et al. | ||
ImageJ | NIH | ||
Flow cytometer | BD | Celesta | |
FACS sorter | BD | Aria III | |
Microscope | Mshot | MF50 | |
Digital Slide Scanners | 3D HISTECH | MIDI | |
XF-96 Extracellular Flux Analyzer | Seahorse Bioscience | N/A | |
Luminometer | GloMax® 20/20 | N/A | |
Confocal microscope | ZEISS | LSM 980 |
- Open table in a new tab
Experimental model and study participant details
Human subjects, primary cell cultures and cell lines, method details, vector construction and lentivirus production, spontaneous liver cancer model, chromatin immunoprecipitation and chip-seq, flow cytometry and cell sorting, immunoblotting, isolation and in vitro treatment of bmdms, mitochondria, fatty acid uptake, and lipid content assays, cellular energy metabolism analysis, luciferase reporter assay, cell proliferation assay, t cell suppression assay, rna-seq and data analysis, dna pull-down, single nucleus rna sequencing (snrna-seq) and data analysis, spatial transcriptomic assay, tumor engraftment, antibody treatment, and macrophage depletion, analysis of tcga/icgc data and public scrna-seq data, statistical analysis, supplemental information (2), article metrics, related articles.
- Download Hi-res image
- Download .PPT
- Cancer Cell
- Cell Chemical Biology
- Cell Genomics
- Cell Host & Microbe
- Cell Metabolism
- Cell Reports
- Cell Reports Medicine
- Cell Reports Methods
- Cell Reports Physical Science
- Cell Stem Cell
- Cell Systems
- Chem Catalysis
- Current Biology
- Developmental Cell
- Molecular Cell
- STAR Protocols
- Biochemical Sciences
- Biotechnology
- Cell Biology
- Cognitive Sciences
- Ecology & Evolution
- Endocrinology & Metabolism
- Microbiology
- Molecular Medicine
- Neurosciences
- Parasitology
- Pharmacological Sciences
- Plant Science
- Biophysical Journal
- Biophysical Reports
- EBioMedicine
- HGG Advances
- Molecular Plant
- Molecular Therapy Family
- Plant Communications
- Stem Cell Reports
- The Innovation
- Submit article
- Multi-Journal Submission
- STAR Methods
- Sneak Peek – Preprints
- Information for reviewers
- Cell Symposia
- Consortia Hub
- Cell Press Podcast
- Cell Press Videos
- Coloring and Comics
- Cell Picture Show
- Research Arc
- About Cell Press
- Open access
- Sustainability hub
- Inclusion and diversity
- Help & Support
- Cell Press Careers
- Scientific job board
- Read-It-Now
- Recommend to Librarian
- Publication Alerts
- Best of Cell Press
- Cell Press Reviews
- Cell Press Selections
- Nucleus Collections
- SnapShot Archive
- For Advertisers
- For Recruiters
- For Librarians
- Privacy Policy
- Terms and Conditions
- Accessibility
The content on this site is intended for healthcare professionals and researchers across all fields of science.
We use cookies to help provide and enhance our service and tailor content. To update your cookie settings, please visit the Cookie settings for this site. All content on this site: Copyright © 2024 Elsevier Inc., its licensors, and contributors. All rights are reserved, including those for text and data mining, AI training, and similar technologies. For all open access content, the Creative Commons licensing terms apply.
- Privacy Policy
- Terms & Conditions
- Accessibility
- Help & Support

Session Timeout (2:00)
Your session will expire shortly. If you are still working, click the ‘Keep Me Logged In’ button below. If you do not respond within the next minute, you will be automatically logged out.
Information
- Author Services
Initiatives
You are accessing a machine-readable page. In order to be human-readable, please install an RSS reader.
All articles published by MDPI are made immediately available worldwide under an open access license. No special permission is required to reuse all or part of the article published by MDPI, including figures and tables. For articles published under an open access Creative Common CC BY license, any part of the article may be reused without permission provided that the original article is clearly cited. For more information, please refer to https://www.mdpi.com/openaccess .
Feature papers represent the most advanced research with significant potential for high impact in the field. A Feature Paper should be a substantial original Article that involves several techniques or approaches, provides an outlook for future research directions and describes possible research applications.
Feature papers are submitted upon individual invitation or recommendation by the scientific editors and must receive positive feedback from the reviewers.
Editor’s Choice articles are based on recommendations by the scientific editors of MDPI journals from around the world. Editors select a small number of articles recently published in the journal that they believe will be particularly interesting to readers, or important in the respective research area. The aim is to provide a snapshot of some of the most exciting work published in the various research areas of the journal.
Original Submission Date Received: .
- Active Journals
- Find a Journal
- Journal Proposal
- Proceedings Series
- For Authors
- For Reviewers
- For Editors
- For Librarians
- For Publishers
- For Societies
- For Conference Organizers
- Open Access Policy
- Institutional Open Access Program
- Special Issues Guidelines
- Editorial Process
- Research and Publication Ethics
- Article Processing Charges
- Testimonials
- Preprints.org
- SciProfiles
- Encyclopedia
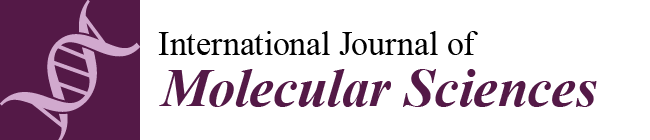
Article Menu
- Subscribe SciFeed
- Recommended Articles
- Google Scholar
- on Google Scholar
- Table of Contents
Find support for a specific problem in the support section of our website.
Please let us know what you think of our products and services.
Visit our dedicated information section to learn more about MDPI.
JSmol Viewer
Three-dimensional-bioprinted non-small cell lung cancer models in a mouse phantom for radiotherapy research.
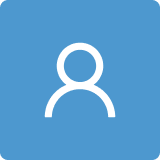
Graphical Abstract
1. Introduction
2.1. development of a 3d-printed human lung cancer model for radiotherapy experiments, 2.2. comparison of dose distributions of the mouse phantom with those of an in vivo mouse model, 2.3. cell viability and metabolic activity of 2d cells and 3d models after radiotherapy, 2.4. radiotherapy induces dna double-strand breaks in 2d cells and 3d models, 2.5. radiotherapy induced apoptosis and ldh release in 2d cells and 3d models, 2.6. influence of the mouse phantom on irradiation effects, 3. discussion, 4. materials and methods, 4.1. cell culture, 4.2. three-dimensional bioprinting, 4.3. model system setup and treatment planning, 4.4. irradiation, 4.5. cell viability assay, 4.6. metabolic activity assay, 4.7. immunofluorescence staining of 2d cells and 3d models, 4.8. cytotoxicity assay, 4.9. statistics, 5. conclusions, supplementary materials, author contributions, institutional review board statement, informed consent statement, data availability statement, acknowledgments, conflicts of interest.
- Siegel, R.L.; Miller, K.D.; Fuchs, H.E.; Jemal, A. Cancer Statistics, 2021. CA-Cancer J. Clin. 2021 , 71 , 7–33. [ Google Scholar ] [ CrossRef ] [ PubMed ]
- Oliver, A.L. Lung Cancer: Epidemiology and Screening. Surg. Clin. N. Am. 2022 , 102 , 335–344. [ Google Scholar ] [ CrossRef ] [ PubMed ]
- Brown, S.; Banfill, K.; Aznar, M.C.; Whitehurst, P.; Faivre Finn, C. The evolving role of radiotherapy in non-small cell lung cancer. Br. J. Radiol. 2019 , 92 , 20190524. [ Google Scholar ] [ CrossRef ] [ PubMed ]
- Meagan, M.; Nasser, H. Advances in systemic therapy for non-small cell lung cancer. BMJ 2021 , 375 , n2363. [ Google Scholar ]
- Vinod, S.K.; Hau, E. Radiotherapy treatment for lung cancer: Current status and future directions. Respirology 2020 , 25 (Suppl. S2), 61–71. [ Google Scholar ] [ CrossRef ]
- Yu, Z.; Xu, C.; Song, B.; Zhang, S.; Chen, C.; Li, C.; Zhang, S. Tissue fibrosis induced by radiotherapy: Current understanding of the molecular mechanisms, diagnosis and therapeutic advances. J. Transl. Med. 2023 , 21 , 708. [ Google Scholar ] [ CrossRef ]
- Ghita, M.; Dunne, V.; Hanna, G.G.; Prise, K.M.; Williams, J.P.; Butterworth, K.T. Preclinical models of radiation-induced lung damage: Challenges and opportunities for small animal radiotherapy. Br. J. Radiol. 2019 , 92 , 20180473. [ Google Scholar ] [ CrossRef ]
- Butterworth, K.T. Evolution of the Supermodel: Progress in Modelling Radiotherapy Response in Mice. Clin. Oncol. (R. Coll. Radiol.) 2019 , 31 , 272–282. [ Google Scholar ] [ CrossRef ]
- Li, Y.; Liu, J.; Xu, S.; Wang, J. 3D Bioprinting: An Important Tool for Tumor Microenvironment Research. Int. J. Nanomed. 2023 , 18 , 8039–8057. [ Google Scholar ] [ CrossRef ]
- De, S.; Singh, N. Advancements in Three Dimensional In-Vitro Cell Culture Models. Chem. Rec. 2022 , 22 , e202200058. [ Google Scholar ] [ CrossRef ]
- Mendes, N.; Dias Carvalho, P.; Martins, F.; Mendonça, S.; Malheiro, A.R.; Ribeiro, A.; Carvalho, J.; Velho, S. Animal Models to Study Cancer and Its Microenvironment. Adv. Exp. Med. Biol. 2020 , 1219 , 389–401. [ Google Scholar ] [ PubMed ]
- Lin, M.; Tang, M.; Duan, W.; Xia, S.; Liu, W.; Wang, Q. 3D Bioprinting for Tumor Metastasis Research. ACS Biomater. Sci. Eng. 2023 , 9 , 3116–3133. [ Google Scholar ] [ CrossRef ] [ PubMed ]
- Marshall, L.J.; Bailey, J.; Cassotta, M.; Herrmann, K.; Pistollato, F. Poor Translatability of Biomedical Research Using Animals—A Narrative Review. Altern. Lab. Anim. 2023 , 51 , 102–135. [ Google Scholar ] [ CrossRef ] [ PubMed ]
- Wegner, M.; Frenzel, T.; Krause, D.; Gargioni, E. Development and characterization of modular mouse phantoms for end-to-end testing and training in radiobiology experiments. Phys. Med. Biol. 2023 , 68 , 085009. [ Google Scholar ] [ CrossRef ] [ PubMed ]
- Al-Zeer, M.A.; Prehn, F.; Fiedler, S.; Lienert, U.; Krisch, M.; Berg, J.; Kurreck, J.; Hildebrandt, G.; Schültke, E. Evaluating the Suitability of 3D Bioprinted Samples for Experimental Radiotherapy: A Pilot Study. Int. J. Mol. Sci. 2022 , 23 , 9951. [ Google Scholar ] [ CrossRef ]
- Jelgersma, C.; Senger, C.; Kluge, A.K.; Janas, A.; Nieminen-Kelhä, M.; Kremenetskaia, I.; Mueller, S.; Brandenburg, S.; Loebel, F.; Tinhofer, I.; et al. Establishment and Validation of CyberKnife Irradiation in a Syngeneic Glioblastoma Mouse Model. Cancers 2021 , 13 , 3416. [ Google Scholar ] [ CrossRef ]
- Hauth, F.; Toulany, M.; Zips, D.; Menegakis, A. Cell-line dependent effects of hypoxia prior to irradiation in squamous cell carcinoma lines. Clin. Transl. Radiat. Oncol. 2017 , 5 , 12–19. [ Google Scholar ] [ CrossRef ]
- LV, B.; Wang, Y.; Ma, D.; Cheng, W.; Liu, J.; Yong, T.; Chen, H.; Wang, C. Immunotherapy: Reshape the Tumor Immune Microenvironment. Front. Immunol. 2022 , 13 , 844142. [ Google Scholar ] [ CrossRef ]
- Mei, Y.; Wu, D.; Berg, J.; Tolksdorf, B.; Roehrs, V.; Kurreck, A.; Hiller, T.; Kurreck, J. Generation of a Perfusable 3D Lung Cancer Model by Digital Light Processing. Int. J. Mol. Sci. 2023 , 24 , 6071. [ Google Scholar ] [ CrossRef ]
- Ojansivu, M.; Rashad, A.; Ahlinder, A.; Massera, J.; Mishra, A.; Syverud, K.; Finne-Wistrand, A.; Miettinen, S.; Mustafa, K. Wood-based nanocellulose and bioactive glass modified gelatin-alginate bioinks for 3D bioprinting of bone cells. Biofabrication 2019 , 11 , 035010. [ Google Scholar ] [ CrossRef ]
- Patrick, P.S.; Bear, J.C.; Fitzke, H.E.; Zaw-Thin, M.; Parkin, I.P.; Lythgoe, M.F.; Kalber, T.L.; Stuckey, D.J. Radio-metal cross-linking of alginate hydrogels for non-invasive in vivo imaging. Biomaterials 2020 , 243 , 119930. [ Google Scholar ] [ CrossRef ] [ PubMed ]
- Berg, J.; Weber, Z.; Fechler-Bitteti, M.; Hocke, A.C.; Hippenstiel, S.; Elomaa, L.; Weinhart, M.; Kurreck, J. Bioprinted Multi-Cell Type Lung Model for the Study of Viral Inhibitors. Viruses 2021 , 13 , 1590. [ Google Scholar ] [ CrossRef ] [ PubMed ]
- Kang, D.; Liu, Z.; Qian, C.; Huang, J.; Zhou, Y.; Mao, X.; Qu, Q.; Liu, B.; Wang, J.; Hu, Z.; et al. 3D bioprinting of a gelatin-alginate hydrogel for tissue-engineered hair follicle regeneration. Acta Biomater. 2023 , 165 , 19–30. [ Google Scholar ] [ CrossRef ] [ PubMed ]
- Hiller, T.; Berg, J.; Elomaa, L.; Röhrs, V.; Ullah, I.; Schaar, K.; Dietrich, A.C.; Al-Zeer, M.A.; Kurtz, A.; Hocke, A.C.; et al. Generation of a 3D Liver Model Comprising Human Extracellular Matrix in an Alginate/Gelatin-Based Bioink by Extrusion Bioprinting for Infection and Transduction Studies. Int. J. Mol. Sci. 2018 , 19 , 3129. [ Google Scholar ] [ CrossRef ]
- Shukla, P.; Yeleswarapu, S.; Heinrich, M.A.; Prakash, J.; Pati, F. Mimicking tumor microenvironment by 3D bioprinting: 3D cancer modeling. Biofabrication 2022 , 14 , 032002. [ Google Scholar ] [ CrossRef ]
- Liu, X.; Jiang, C.; Zhang, D.; Gao, M.; Peng, F.; Huang, D.; Sun, Z.; Ni, Y.; Zhang, J.; Yin, Z. Tumor necrosis targeted radiotherapy of non-small cell lung cancer using radioiodinated protohypericin in a mouse model. Oncotarget 2015 , 6 , 26400–26410. [ Google Scholar ] [ CrossRef ]
- Frenzel, T.; Hoffmann, B.; Schmitz, R.; Bethge, A.; Schumacher, U.; Wedemann, G. Radiotherapy and chemotherapy change vessel tree geometry and metastatic spread in a small cell lung cancer xenograft mouse tumor model. PLoS ONE 2017 , 12 , e0187144. [ Google Scholar ] [ CrossRef ]
- Pustovalova, M.; Alhaddad, L.; Smetanina, N.; Chigasova, A.; Blokhina, T.; Chuprov-Netochin, R.; Osipov, A.N.; Leonov, S. The p53-53BP1-Related Survival of A549 and H1299 Human Lung Cancer Cells after Multifractionated Radiotherapy Demonstrated Different Response to Additional Acute X-ray Exposure. Int. J. Mol. Sci. 2020 , 21 , 3342. [ Google Scholar ] [ CrossRef ]
- Chen, J.L.; Pan, C.K.; Lin, Y.L.; Tsai, C.Y.; Huang, Y.S.; Yang, W.C.; Hsu, F.M.; Kuo, S.H.; Shieh, M.J. Preclinical evaluation of PEGylated liposomal doxorubicin as an effective radiosensitizer in chemoradiotherapy for lung cancer. Strahlenther. Onkol. 2021 , 197 , 1131–1142. [ Google Scholar ] [ CrossRef ]
- Chen, J.L.; Pan, C.K.; Lin, L.C.; Tsai, C.Y.; Kuo, C.Y.; Huang, Y.S.; Lin, Y.L. Therapeutic efficacy of cyclin-dependent kinase inhibition in combination with ionizing radiation for lung cancer. Int. J. Radiat. Biol. 2023 , 99 , 1257–1266. [ Google Scholar ] [ CrossRef ]
- Beckers, C.; Pruschy, M.; Vetrugno, I. Tumor hypoxia and radiotherapy: A major driver of resistance even for novel radiotherapy modalities. Semin. Cancer Biol. 2024 , 98 , 19–30. [ Google Scholar ] [ CrossRef ] [ PubMed ]
- Mitrakas, A.G.; Giatromanolaki, A.; Koukourakis, M.I. Hypoxia and acidity regulate immune checkpoint molecule and IFN-β expression in non-small cell lung cancer cell lines. J. Recept. Signal Transduct. Res. 2023 , 43 , 31–36. [ Google Scholar ] [ CrossRef ] [ PubMed ]
- Wu, D.; Berg, J.; Arlt, B.; Röhrs, V.; Al-Zeer, M.A.; Deubzer, H.E.; Kurreck, J. Bioprinted Cancer Model of Neuroblastoma in a Renal Microenvironment as an Efficiently Applicable Drug Testing Platform. Int. J. Mol. Sci. 2022 , 23 , 122. [ Google Scholar ] [ CrossRef ] [ PubMed ]
- Wu, D.; Pang, S.; Röhrs, V.; Berg, J.; Ali, A.S.M.; Mei, Y.; Ziersch, M.; Tolksdorf, B.; Kurreck, J. Man vs. machine: Automated bioink mixing device improves reliability and reproducibility of bioprinting results compared to human operators. Int. J. Bioprint. 2024 , 10 , 380. [ Google Scholar ] [ CrossRef ]
Click here to enlarge figure
The statements, opinions and data contained in all publications are solely those of the individual author(s) and contributor(s) and not of MDPI and/or the editor(s). MDPI and/or the editor(s) disclaim responsibility for any injury to people or property resulting from any ideas, methods, instructions or products referred to in the content. |
Share and Cite
Mei, Y.; Lakotsenina, E.; Wegner, M.; Hehne, T.; Krause, D.; Hakimeh, D.; Wu, D.; Schültke, E.; Hausmann, F.; Kurreck, J.; et al. Three-Dimensional-Bioprinted Non-Small Cell Lung Cancer Models in a Mouse Phantom for Radiotherapy Research. Int. J. Mol. Sci. 2024 , 25 , 10268. https://doi.org/10.3390/ijms251910268
Mei Y, Lakotsenina E, Wegner M, Hehne T, Krause D, Hakimeh D, Wu D, Schültke E, Hausmann F, Kurreck J, et al. Three-Dimensional-Bioprinted Non-Small Cell Lung Cancer Models in a Mouse Phantom for Radiotherapy Research. International Journal of Molecular Sciences . 2024; 25(19):10268. https://doi.org/10.3390/ijms251910268
Mei, Yikun, Elena Lakotsenina, Marie Wegner, Timon Hehne, Dieter Krause, Dani Hakimeh, Dongwei Wu, Elisabeth Schültke, Franziska Hausmann, Jens Kurreck, and et al. 2024. "Three-Dimensional-Bioprinted Non-Small Cell Lung Cancer Models in a Mouse Phantom for Radiotherapy Research" International Journal of Molecular Sciences 25, no. 19: 10268. https://doi.org/10.3390/ijms251910268
Article Metrics
Article access statistics, supplementary material.
ZIP-Document (ZIP, 752 KiB)
Further Information
Mdpi initiatives, follow mdpi.
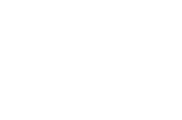
Subscribe to receive issue release notifications and newsletters from MDPI journals
Thank you for visiting nature.com. You are using a browser version with limited support for CSS. To obtain the best experience, we recommend you use a more up to date browser (or turn off compatibility mode in Internet Explorer). In the meantime, to ensure continued support, we are displaying the site without styles and JavaScript.
- View all journals
- Explore content
- About the journal
- Publish with us
- Sign up for alerts
- Open access
- Published: 28 September 2024
Insight into mammary gland development and tumor progression in an E2F5 conditional knockout mouse model
- Briana To 1 ,
- Carson Broeker ORCID: orcid.org/0000-0003-3621-2884 1 ,
- Jing-Ru Jhan 1 ,
- Jesus Garcia-Lerena 1 ,
- John Vusich 1 ,
- Rachel Rempel 2 ,
- Jonathan P. Rennhack 1 ,
- Daniel Hollern 3 ,
- Lauren Jackson 1 ,
- David Judah 1 na1 ,
- Matt Swiatnicki 1 ,
- Evan Bylett 1 ,
- Rachel Kubiak 1 ,
- Jordan Honeysett 1 ,
- Joseph Nevins 2 &
- Eran Andrechek 1
Oncogene ( 2024 ) Cite this article
Metrics details
- Breast cancer
- Cancer models
Development of breast cancer is linked to altered regulation of mammary gland developmental processes. A better understanding of normal mammary gland development can thus reveal possible mechanisms of how normal cells are re-programmed to become malignant. E2Fs 1-4 are part of the E2F transcription factor family with varied roles in mammary development, but little is known about the role of E2F5. A combination of scRNAseq and predictive signature tools demonstrated the presence of E2F5 in the mammary gland and showed changes in predicted activity during the various phases of mammary gland development. Testing the hypothesis that E2F5 regulates mammary function, we generated a mammary-specific E2F5 knockout mouse model, resulting in modest mammary gland development changes. However, after a prolonged latency the E2F5 conditional knockout mice developed highly metastatic mammary tumors. Whole genome sequencing revealed significant intertumor heterogeneity. RNAseq and protein analysis identified altered levels of Cyclin D1, with similarities to MMTV-Neu tumors, suggesting that E2F5 conditional knockout mammary glands and tumors may be dependent on Cyclin D1. Transplantation of the tumors revealed metastases to lymph nodes that were enriched through serial transplantation in immune competent recipients. Based on these findings, we propose that loss of E2F5 leads to altered regulation of Cyclin D1, which facilitates the development of metastatic mammary tumors after long latency. More importantly, this study demonstrates that conditional loss of E2F5 in the mammary gland leads to tumor formation, revealing its role as a transcription factor regulating a network of genes that normally result in a tumor suppressor function.
Similar content being viewed by others
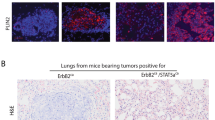
STAT5 confers lactogenic properties in breast tumorigenesis and restricts metastatic potential
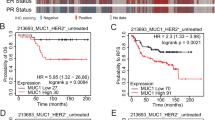
MUC1 triggers lineage plasticity of Her2 positive mammary tumors
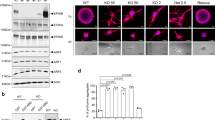
EFA6B regulates a stop signal for collective invasion in breast cancer
The mammary gland is a complex organ that undergoes dynamic changes during different stages of development. Analysis of transcriptional profiles at each developmental stage, including pregnancy, lactation and involution, have revealed unique gene expression changes [ 1 , 2 ]. One family of transcription factors that regulates these intricate transcriptional changes are the E2F transcription factors. The E2F transcription factor family consists of 8 members that are routinely divided to transcriptional activators and repressor [ 3 , 4 , 5 , 6 ] and are best known for their role in cell cycle progression [ 7 , 8 , 9 , 10 ]. However, they are a functionally diverse group of transcription factors with numerous studies highlighting their role in apoptosis [ 11 ], cell differentiation [ 12 , 13 ], metabolism [ 14 ] and development [ 15 , 16 , 17 , 18 , 19 ]. The role of E2Fs in development was established through the characterization of single and compound E2F knockout mice. Following that, the role of E2Fs in mammary gland development was characterized in single knockouts of E2F1-4 [ 20 ]. Loss of E2F1, E2F3 or E2F4 resulted in mammary outgrowth delay and branching defects. However, these changes were not observed in E2F2 knockout mice. Given the high functional redundancy observed among E2Fs [ 21 ], the extent of compensation between the activator E2Fs in the mammary gland was explored. Using double knockout mice, E2F2 was found to partially compensate for the loss of E2F1 but not E2F3 in mammary ductal outgrowth, and transplant experiments demonstrated that these were cell autonomous effects [ 22 ].
E2F regulated functions are also integral in breast cancer development and progression to metastatic disease. For instance, specific activator E2Fs have unique roles in mediating metastasis, roles that are dependent upon the context of the activating oncogene with noted differences in tumors driven by Myc, Neu or PyMT [ 23 , 24 , 25 , 26 , 27 ]. E2Fs transcriptionally regulate a broad range of targets that are involved in many cancer associated pathways including apoptosis, genomic instability and DNA damage response [ 28 ]. Furthermore, E2Fs also have the potential to regulate oncogenes, with well characterized effects both up and downstream of Myc [ 6 , 29 , 30 , 31 ]. The role of the E2Fs in development of the mammary gland and in altering tumor biology have been described for the activator E2Fs and repressor E2F4. However, the main E2F repressors include both E2F4 and E2F5 and the role of E2F5 in mammary development is not known. Similar to E2F4, E2F5 is considered to be a transcriptional repressor and canonically functions to repress cell cycle progression [ 32 , 33 ]. Furthermore, E2F4 and E2F5 share the most structural similarities among all the E2F members and have demonstrated functional redundancy [ 32 ]. The role of E2F5 in tumorigenesis is conflicted and unclear as the literature for E2F5 in cancer biology reveals some studies suggesting an inhibition of transformation [ 34 ] while others note an oncogenic role [ 35 , 36 , 37 ]. Given the wide variety of target genes regulated by the E2Fs, it is likely that the role of E2F5 is largely tissue, cell type and context dependent, with roles that widely differ in tissue and tumor types. E2F5 has been understudied in part due to the early lethality associated with the knockout which results in hydrocephaly in prepubertal mice [ 19 ], making phenotypic examination and experimental manipulation more difficult. Interestingly, the hydrocephaly is distinct and is not observed in E2F4 knockout mice [ 18 ] but both E2Fs have functional overlap and combine to contribute to cell cycle regulation [ 32 ].
Here we have hypothesized that E2F5 plays an essential role in mammary gland development. To test this prediction, we generated mice with a conditional ablation of E2F5 in the mammary gland. In these mice we observed alterations to development and after a long latency, formation of metastatic tumors. Importantly, the transplantable tumors from these mice develop lymph node metastases, a trait not widely reported for other genetically engineered mouse models of breast cancer.
To begin to investigate a potential role for E2F5 in mammary development and function, we examined a scRNAseq dataset [ 38 ] that was clustered into the various stages of mammary development, including nulliparious, pregnant, lactating and involuting (Fig. 1A ). As a control, we first examined this scRNAseq data for E2F1 expression (Fig. 1B ) and noted expected expression in the nulliparous and early pregnancy stages. We then examined this data for E2F5 expression (Fig. 1C ), it revealed the highest level of expression of E2F5 in the lactating and involuting cells. Given the expression in different cell types we assessed E2F5 expression in a survey of various cell types within the mammary gland [ 39 ], using an involuting gland to have good representation of immune cells during remodeling (Fig. 1D ). This revealed that E2F5 expression was present in several cell types, which Han et al. identified through various markers, including secretory alveoli as well as endothelial cells and fibroblasts (Fig. 1E and Supplemental Fig. 1 ). Interestingly, a comparison of differentiation states from the Bach et al. data revealed that E2F5 was expressed in progenitor cells as well as the differentiated secretory and hormone sensing lineages, a sharp contrast to the E2F1, a typical activator E2F (Fig. 1F ). While expression of E2F5 leads to plausible hypotheses about function, expression is not linked to activity. To assess this, we generated a signature for E2F5 activation by overexpressing E2F5 using an adenoviral vector in Human Mammary Epithelial Cells (HMECs), with increasing multiplicity of infection tied to E2F5 levels (Fig. 1G ). After infection, RNA was isolated after 18 h to generate a gene expression signature using microarrays. HMECs infected with the E2F5 construct were compared to controls with a GFP construct and a signature was generated using a Bayesian approach [ 40 ]. Despite being classically known as a transcriptional repressor, E2F5 expression resulted in both upregulated and downregulated genes, suggesting that E2F5 may also play a role as transcriptional activator [ 32 , 33 ]. The up and down regulated genes in the signature were then tested for their predictive activity on a series of human breast cancer cell lines where predicted activity was compared to protein levels, validating the signature (Fig. 1H ). A mammary gland developmental dataset [ 1 ] was then limited to the E2F5 signature genes and clustered, revealing that E2F5 regulated genes stratified mammary developmental stages (Fig. 1I ). In addition, we characterized the subset of E2F5 regulated genes enriched in each developmental stage using gene ontology (Supplemental table 1 ). In the virgin and early pregnancy stages from Fig. 1I , the up and down regulated genes expressed after E2F5 overexpression were associated with various ontologies. For example, the most significant ontology in virgin and early pregnancy was organelle transport along microtubules (Supplemental Table 1 ). Later pregnancy timepoints were seen to have apoptotic processes enriched. However, as shown in data below, loss of E2F5 does not appreciably alter lactation, generating questions as to why apoptotic pathways were observed in the late pregnancy data. Next, we tested genes with a fold change in a terminal end bud/duct dataset [ 41 ] and found that nearly 10% of terminal end bud genes were also E2F5 regulated genes (Supplemental Fig. 2 ). Thus, we hypothesized that E2F5 played both developmental and functional roles in the mouse mammary gland.
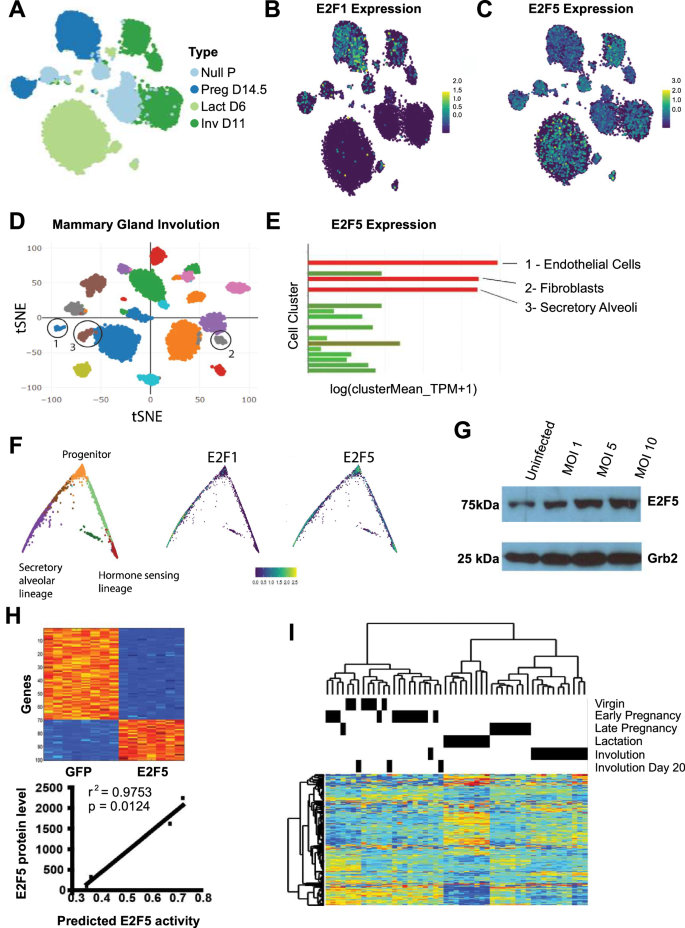
Using a mouse mammary scRNAseq dataset that was split to various functional stages ( A ) including nulliparous (Null P) pregnancy day 14.5 (Preg D14.5), lactation day 6 (Lact D6) and involution day 11 (Inv D11). For each stage, the level of E2F1 ( B ) and E2F5 ( C ) expression was plotted. Using a separate scRNAseq dataset that was not sorted for epithelial cells ( D ), expression of E2F5 was examined across cell populations in the involuting mammary gland, revealing expression in endothelial cells, fibroblasts and alveoli with elevated signal in red and lower signaling in green ( E ). Examining lineage commitment ( F ), we overlaid E2F1 and E2F5 expression with elevated expression in green. To generate a signature for E2F5 activity, HMECs were infected with increasing multiplicity of infection (MOI) for an adenovirus expressing GFP or E2F5. A western blot demonstrated increasing levels of E2F5 with increasing MOI ( G ). Generation of a signature for E2F5 activation revealed genes up (orange/red) and down (blue) regulated. The activity of the signature was predicted in several human breast cancer cell lines and was plotted against the observed levels in a western blot for E2F5 revealing correlation between the two ( H ). A mammary gland developmental dataset (Stein et al., 2004) was limited to the E2F5 signature genes and was clustered, revealing that genes regulated by E2F5 stratified mammary developmental stages ( I ).
To directly test the role of E2F5 in mammary development we sought to use a knockout mouse model. With the lethality observed in the global knockout [ 19 ], we generated a strain of mice with loxP sites flanking exons 2 and 3 of E2F5 (Fig. 2A , Supplemental Fig. 3A, B ). Through breeding to the MMTV-Cre strain [ 42 ] with expression limited to mammary epithelium [ 43 ], we generated a mammary specific knockout of E2F5 (Fig. 2A ). Prior to interbreeding with MMTV-Cre transgenics, the E2F5 flox/flox mice were backcrossed into the FVB background for 12 generations. Introduction of MMTV-Cre to the E2F5 flox/flox strain resulted in the expected incomplete excision, which was not complete since whole mammary glands were tested and MMTV directed Cre expression is limited to mammary epithelium (Supplemental Fig. 3C ). Testing for a developmental role, we examined mammary gland outgrowth at 4 weeks of age where the littermate controls had terminal end buds that had driven outgrowth past the lymph node in the fat pad (Fig. 2D ). The E2F conditional knockouts (E2F5 CKO) had delayed development (Fig. 2E ). Quantification of these results revealed a consistent delay in ductal extension (Fig. 2F , p = 0.0019). Heterozygous mice were not examined since the delay was slight. Other stages of mammary gland function were also assayed, with lactation occurring normally as assessed by histology and pup weight (Supplemental Fig. 4 ). To test for effects in older virgin mice, we allowed a cohort of controls and E2F5 CKO mice to age to 12 months. This revealed standard development in the controls and surprising alveolar overgrowth in the virgin E2F5 CKO mice (Fig. 2G, H ).
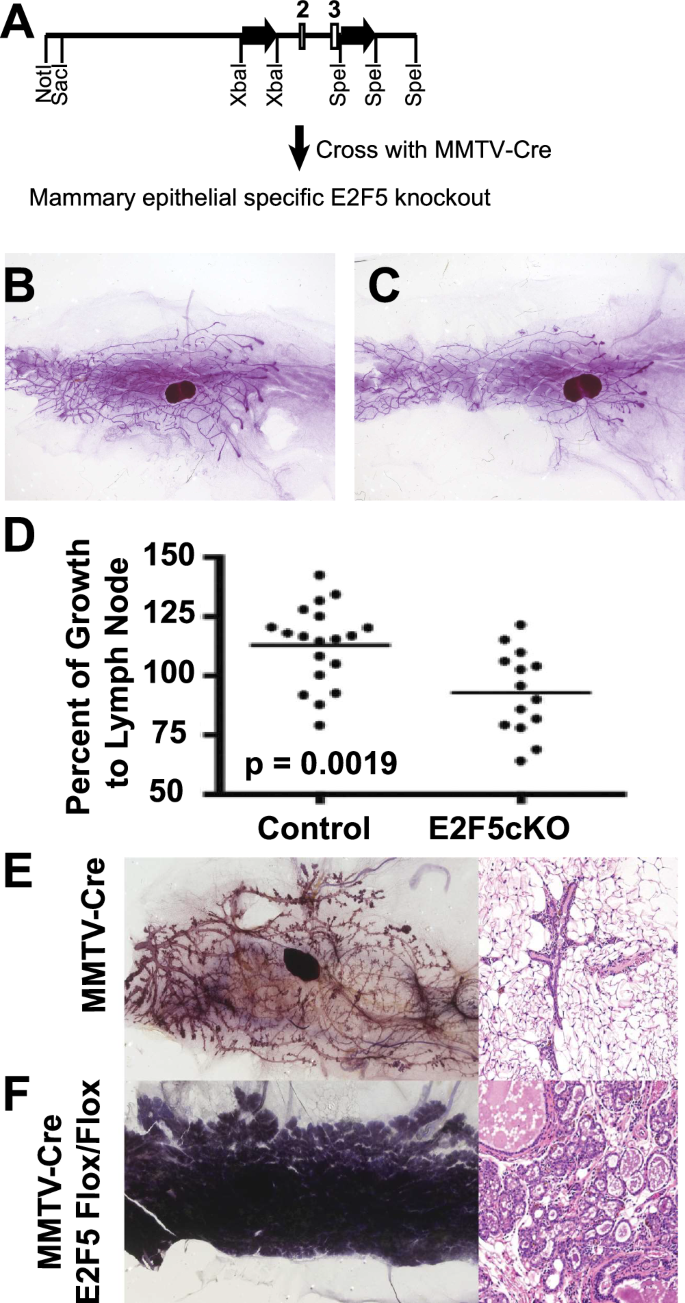
A gene targeting strategy to flank exons 2 and 3 of E2F5 with loxP sites was employed with genotyping primers shown ( A ). With the introduction of Cre Recombinase under the mammary epithelial specific control of the MMTV promoter/enhancer, exons 2 and 3 are lost resulting in a tissue specific knockout. Examining ductal extension through wholemounts at 4 weeks we examined 20 control mice (E2F5 flox/flox ) ( B ) and 14 E2F5 CKO mice ( C ), revealing a delay in outgrowth. This delay was quantified revealing a consistent outgrowth delay, 0 = 0.0019 ( D ). After mice aged to 12 months, virgin mammary glands were assessed by both wholemount and histology. Relative to the MMTV-Cre controls ( E ) with their somewhat spiked ductal appearance, the E2F5 CKO mammary glands resembled a lactating mammary gland with alveoli engulfing the entire fat pad ( F ).
With the overgrowth of the virgin mammary gland, we carefully observed the E2F5 CKO mice for possible mammary tumor development. After a long latency, virgin E2F5 CKO mice developed focal mammary tumors while the MMTV-Cre control line did not (Fig. 3A ). Multiparous E2F5 CKO mice were noted to have a slight reduction in tumor latency, potentially due to more widespread Cre expression during lactation. Given that MMTV is hormonally responsive, Cre is expressed at a much higher level [ 42 ], resulting in more widespread mammary epithelial E2F5 knockout, and tumors that develop slightly more rapidly. Time to 50% tumor burden for each genotype was as follows: Cre NA, Multiparous 596 days, Virgin 681 days, n = 16, 34 and 44 respectively with 16, 15 and 29 censored data points. Using a log rank test, the difference between virgin and multiparous was significant with p = 0.003. The heterozygous conditional deletion mice were not monitored for tumor development, but no MMTV-Cre E2F5 flox/wild type mice were noted to develop a tumor over the time they were used for breeding purposes.
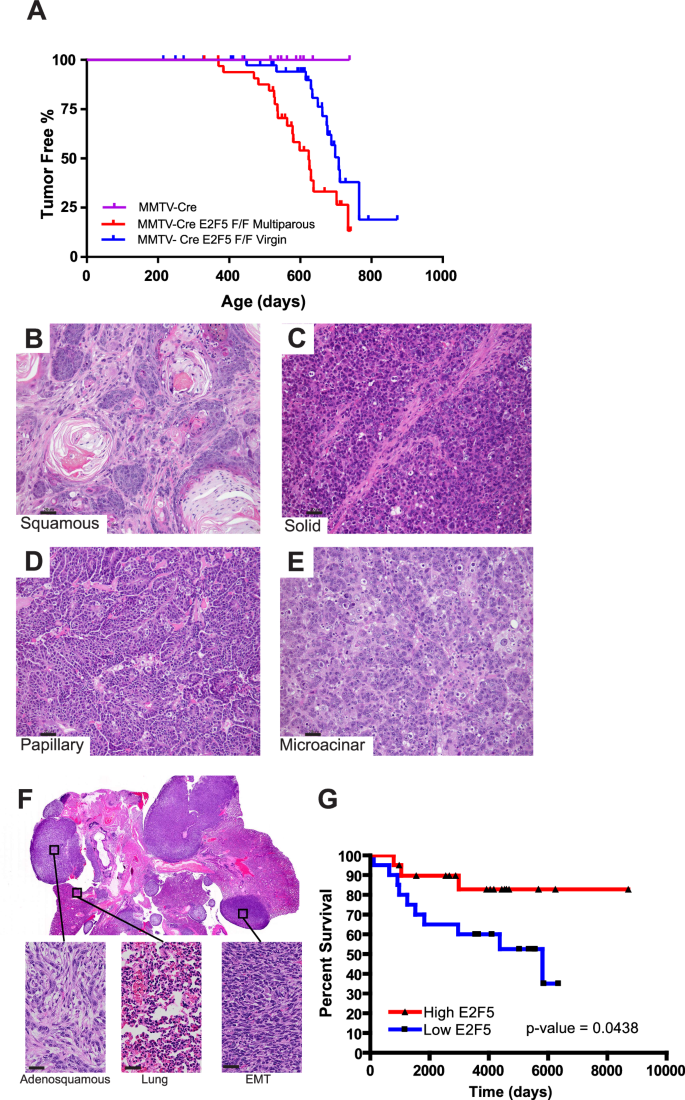
Regular palpation of the mammary glands revealed the onset of tumor formation in E2F5 CKO virgin and multiparous mice, but not in the control MMTV-Cre line ( A ). The resulting tumors were histologically diverse with numerous patterns noted, including squamous ( B ), solid with collagen tracks ( C ), papillary ( D ) and microacinar ( E ). 20-micron scale bars are included. The tumors were also metastatic, a pulmonary section reveals numerous metastatic lesions at both low and high power ( F ), this includes adenosquamous (left panel), normal lung (center panel) and EMT (right panel). Applying the E2F5 signature to human breast cancer stratified the patients to high/low quartiles. These results were consistent with the mouse model as low E2F5 activity was associated with worse survival ( G ).
The resulting tumors exhibited varied histological patterns, with a broad spectrum of subtypes reminiscent of those noted in other heterogenous strains such as MMTV-PyMT [ 25 , 44 ]. Indeed, many of the same histopathologies previously noted in the PyMT and Myc strains were present, including adenosquamous (Fig. 3B ), solid with collagen tracks (Fig. 3C ), papillary (Fig. 3D and microacinar (Fig. 3E ). No appreciable differences in pathology were noted in tumors from virgin and multiparous mice. Several tumors were tested by IHC for both ER and PR and were uniformly negative. CK8 and CK5 IHC staining revealed a mosaic pattern, suggesting both basal and luminal characteristics depending on the tumor (Supplemental Fig. 5 ). These tumors were highly metastatic with 74% of tumor bearing mice developing metastasis, with an average of 2.31 pulmonary metastases detected in a single histological section across the lobes of the lungs. An example of the pulmonary metastases is shown with several regions of the lungs magnified, illustrating that metastases in the lung can contain different pathologies for each metastatic lesion (Fig. 3F with adenosquamous (left panel), normal lung (center panel) and EMT (right panel)). In addition, liver metastasis was occasionally noted in the tumor bearing mice (4 metastases in 33 livers examined). Other metastatic lesions were infrequently noted on the thoracic wall, in the lymph nodes and in the intraperitoneal cavity as shown in Supplemental Fig. 6 . No differences were noted in metastasis rates between virgin and multiparous tumors and it is unclear why only 74% of tumors were metastatic. With loss of E2F5 resulting in tumor formation in the mouse model, we hypothesized that E2F5 function may be related to human breast cancer development or clinical outcomes. Testing the E2F5 signature in a human breast cancer dataset allowed us to stratify patients to high/low quartiles of E2F5 activity. Survival analysis of these patients revealed that low E2F5 activity was associated with a reduction in survival rates (Fig. 3G ). Moreover, at the single gene level, low levels of E2F5 expression in HER2 and triple negative subtypes of breast cancer was linked to worse outcomes (Supplemental Fig. 7A–D ) [ 45 ]. Taken together, these data suggest that E2F5 expression and activity normally has a protective role in human breast cancer.
To explore the mechanisms by which E2F5 may regulate tumor development and progression we used an integrated approach by analyzing genomic and transcriptomic data. We examined whole genome sequence from five E2F5 CKO tumors with diverse histopathology (Supplemental Fig. 8 ). A representative circos plot for one of the tumors is shown with chromosomes around the periphery (Fig. 4A ) with the remainder in supplemental data (Supplemental Fig. 9A–D , Supplemental Fig. 10A–E ). Starting from the outermost region of the plot, an ideogram with labelled mouse chromosomes is provided. Single nucleotide variants (SNV) are shown as points with color reflecting putative impact, followed by copy number variants (CNV) with deletions and amplifications shown with color blocks. In the center, translocations and inversions are shown with lines (Fig. 4A ). The differences between the circos plots were readily evident when comparing circos plots (Supplemental 9A-D). The combined SNV and CNV events impacting at least three tumors are shown (Fig. 4B ), with some genes having more than one event per gene. To directly compare both shared and unique CNV events, we generated a graph demonstrating the CNV events found in the 5 tumors (Fig. 4C ). All CNVs are listed in Supplemental Table 2 . The CNV analysis revealed a number of regions that were partially conserved. A closer examination of chromosome 6 revealed that three of the five tumors shared an amplification event that encompasses Wnt2, Cav1 and Met (Fig. 4D ). The boxed region in Fig. 4D is seen in expanded form in Fig. 4E , where the black line shows the diploid level and the amplification extent in three tumors is evident. Other regions of interest were noted on chromosome 3 and 8, where SNVs were enriched. Numerous SNVs in genes of potential interest were noted in multiple tumors, including in genes such as FBXO15 (5 of 5 tumors) and TSHZ1 (4 of 5 tumors). Other SNVs were highly impactful but were only noted in individual tumors, include p53, KRas, NUDT7 and MTRF1. SNVs that were visually inspected in the sequence data are included in Supplemental Table 2 . Comparison to other WGS data for PyMT and Neu tumors revealed divergence between the tumor genotypes at the SNV level, with the most shared SNVs being between Neu and E2F5 CKO tumors (Supplemental Fig. 11 ). Examining the mutational spectrum of the tumors using the COSMIC SBS mutation signatures (Fig. 4F ), revealed aging and HRR Deficient as the predominant signatures in the E2F5 CKO tumors. It should be noted that the aging signature may be present simply due to the age of the mice at which tumors developed.

Whole genome sequencing on five E2F5 CKO tumors were generated. Analysis of WGS data revealed CNVs, SNVs and translocation for each tumor. An example of a Circos plot generated for a single tumor provides a bird eye view of the genetic alteration identified ( A ). Starting from the outermost region of each plot, an ideogram with labelled mouse chromosomes is first, followed by SNVs at the next innermost ring. SNVs were marked as low (yellow), moderate (orange), and high (red) predicted impact as defined by Mutect2 annotation. The next innermost ring contains all predicted CNVs as defined by the consensus of Delly and Lumpy; deletions are colored blue and duplications are colored red. Within each tumor, the height of CNVs are scaled relative to the CNV with the largest amount of evidence supporting it as determined by Lumpy annotation (e.g. a duplication with 40 pieces of evidence will only be half the height of a deletion with 80 pieces of evidence). The width of each CNV is determined by the start and stop positions determined by the consensus of Delly and Lumpy on the length of the genome. Duplications point outward while deletions point inward starting from a shared midpoint. The innermost ring contains predicted high impact translocations and high to moderate impact inversions by the consensus of Delly and Lumpy calls. Inversions are colored black. Translocation color matches the ideogram color of one of the two chromosomes involved in the event. For all CNV and SNVs found in at least 3 tumors an oncoprint style plot was generated with SNV/CNV per tumor shown above and the SNV/CNV per gene shown at right. For each tumor sample in each column, only the high confidence calls are presented ( B ). Copy number alterations found in the tumors are illustrated with each column representing a chromosome location ( C ). Red indicates amplification while blue indicates deletion. A closer examination at the CNVs located on Chromosome 6 revealed some shared events between the tumors ( D ). The boxed region in ( D ) is expanded for the three tumors containing an amplification at that point in panel E. The COSMIC SBS mutation signatures enriched among the tumors were identified and are presented in panel F.
In addition to the WGS characterization, we also examined the transcriptional profiles of E2F5 CKO tumors through bulk RNAseq on samples derived from whole MMTV-Cre mammary glands (aged 635, 546 and 537 days), whole E2F5 CKO mammary glands (aged 470, 482 and 580 days), E2F5 CKO mammary tumors from all observed histological subtypes and tumor cell lines derived from the E2F5 CKO tumors (E2F5CKO tumor cell lines). First, we performed differential gene expression analysis between whole MMTV-Cre mammary glands and E2F5 CKO mammary glands as well as with E2F5 CKO tumors using the RNA-seq data. After filtering out differentially expressed genes with a fold change <2, the resulting lists of genes were further filtered based on its percent altered in human breast cancer (cut off >5%). The resulting upregulated and downregulated genes were analyzed using Enrichr, a gene set enrichment analysis tool [ 46 ]. The analysis revealed that the upregulated genesets are enriched for genes that are putative targets of E2F4 and E2F6 (Fig. 5A ). Although this may not be surprising as there may be compensation, it does suggest that these differentially expressed genes are likely direct E2F targets and are dysregulated in E2F5CKO tumors. Given that this is a new model, we tested for relation to the known PAM50 subtypes, demonstrating that the tumors aligned with a mixture of Basal, Luminal A and HER2+ve tumors (Supplemental Fig. 12 ). Geneset enrichment analysis on the downregulated genes revealed enrichment for putative targets such as PPARG, SUZ12, MYDO1 and ESR1. (Supplemental Fig. 13 )). Pathway analysis revealed that the upregulated geneset were enriched for pathways associated with cell cycling, Fanconi anemia, DNA repair, and DNA replication signaling pathways with loss of E2F5 (Fig. 5B ). In contrast, the downregulated genesets were enriched for processes involved in normal metabolism (Supplemental Figure 13 ). We then examined the differentially expressed genes in StringDb to visualize which genes have known or predicted interactions with E2F5 (Fig. 5C ). To further narrow down our target gene population, we examined the level of gene expression in both E2F5CKO mammary gland and tumor cell lines. Notably, we observed that Cyclin D1 transcripts were elevated in E2F5CKO mammary glands and significantly elevated in E2F5CKO tumor cell lines (Fig. 5D ). Given that Cyclin D1 appears to be elevated in pre-tumor E2F5 CKO mammary glands, we hypothesize that alteration of Cyclin D1 expression may be critical for tumor formation in E2F5CKO tumors. In line with the differential gene expression analysis, Cyclin D1 and several other genes demonstrated increased expression in E2F5CKO mammary glands and tumors relative to MMTV-Cre mammary glands through qRT-PCR (Supplemental Fig. 14A–E ). However, the most striking difference was seen in Cyclin D1 where there was a significant increase in E2F5CKO tumors relative to control in the RNAseq data (Fig. 5D ), confirmed with a 15-fold increase in Cyclin D1 levels observed through qRT-PCR (Fig. 5E ). To examine whether Cyclin D1 potentially has a prominent role in E2F5 tumors, we analyzed the levels of Cyclin D family members in E2F5CKO tumor in comparison to Wnt-1 and Neu tumors. This revealed that levels of Cyclin D1 were consistent in all three models. However, only cyclin D2 was detected in Wnt-1 tumors (Fig. 6F ). The E2F5 CKO tumors resembled the MMTV-Neu tumors for expression of Cyclin D1, D2 and D3, suggesting that like MMTV-Neu the E2F5 CKO tumors were dependent on Cyclin D1. To further examine the potential inverse relationship between E2F5 and Cyclin D1, we examined Cyclin D1 levels in the setting of E2F5 overexpression in HMECs. Consistent with our previous findings, we found that Cyclin D1 is downregulated in E2F5 overexpressing HMECs that were used in the E2F5 signature creation (Supplemental Fig. 14F ).

Analysis of differentially upregulated genes in E2F5KO using EnRichR on whole mammary glands lacking E2F5 relative to E2F5 CKO tumors revealed putative targets ( A ) and biological pathways ( B ). Further analysis with StringDb revealed the predicted interactions between E2F5 and the differentially upregulated genes ( C ). Using a filtering strategy to determine genes involved with E2F5 CKO tumor formation, we identified Cyclin D1. Comparison of mammary gland expression of cyclin D1 through RNAseq revealed an upregulation in whole mammary glands, tumors and more extensively in the cell lines derived from the tumors ( D ). Validation of these results through qRT-PCR for cyclin D1 in 3 MMTV-Cre and 3 E2F5 CKO whole mammary glands revealed a 15 fold upregulation of cyclin D1 in the pre-tumor mammary glands ( t -test P -value 0.077) ( E ). Examination of Wnt1, Neu and E2F5 CKO tumors revealed that each strain had elevated Cyclin D1 but only Wnt1 tumors had upregulation of Cyclin D2 ( F ). Indeed, the E2F5 CKO tumors closely resembled the MMTV-Neu tumors with elevated Cyclin D1 and lower levels of both Cyclin D2 and D3 (quantification in Supplemental Table 4 ).
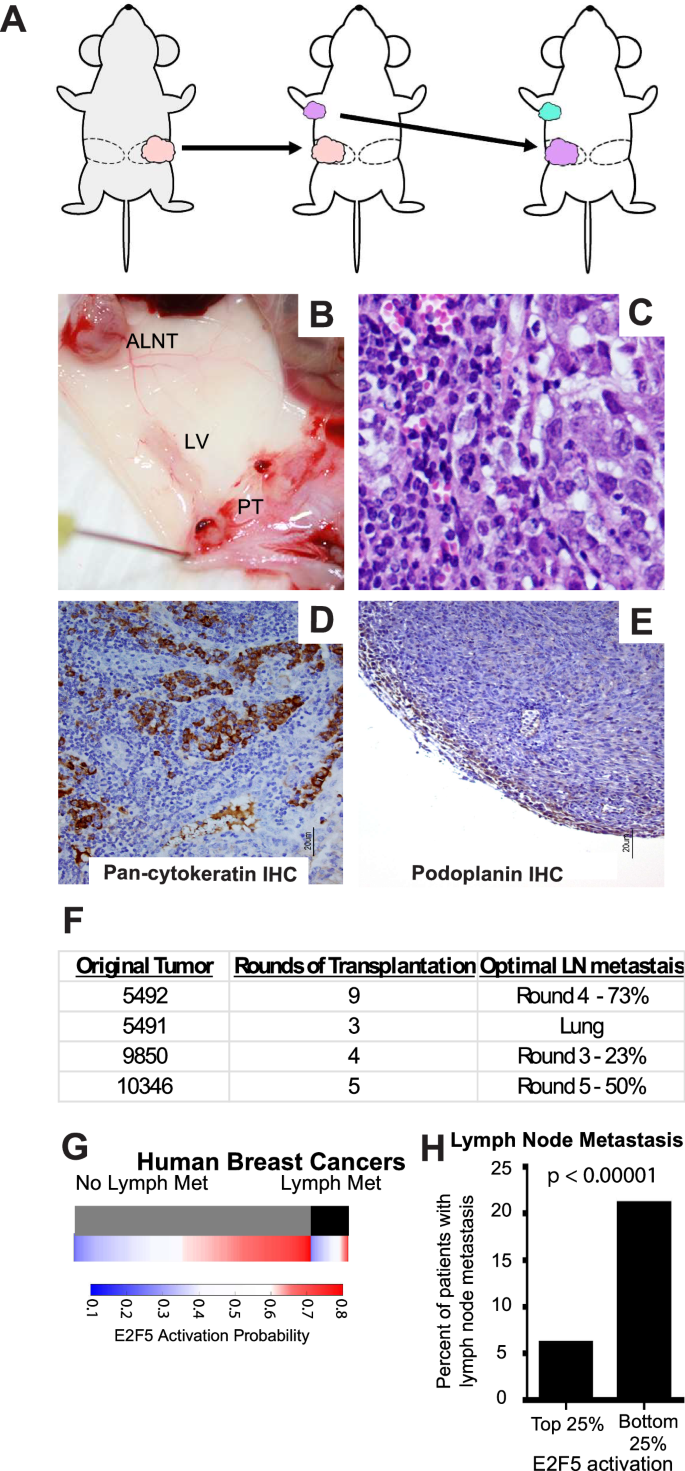
Implantation of E2F5 CKO tumors into FVB MMTV-Cre recipients resulted in mammary and axillary tumor formation. The strategy for serial transplantation of E2F5 CKO axillary tumors into the abdominal mammary gland to enrichlymphatic metastasis is shown ( A ). In an example of an enrichment necropsy, the primary tumor has been excised (abdominal gland, bottom of panel) but a tumor has also formed in the region of the axial lymph node (top) ( B ) Labels include ALNT (axial lymph node tumor), LV (enlarged lymphatic vessel) and the remnants of the excised primary tumor (PT) in the abdominal gland. Cross section of the lymph node reveals both lymph (left side) tissue and metastatic (right side) cells ( C ). Staining with a pan-cytokeratin antibody reveals nests of metastatic cells throughout the lymph node ( D ). Cross section and staining of the enlarged vessel from ( B ) for podoplanin reveals a positively staining lymphatic vessel containing counterstained tumor cells ( E ). A summary for four transplanted lines shows the number of rounds of transplantation and where the optimal lymph node enrichment point was observed with the percent of tumor bearing mice with lymph node metastasis included for each ( F ). Examining human breast cancer with known lymph node status for the E2F5 activation gene signature revealed that metastatic tumors had lower E2F5 activity ( G ). Splitting the samples into quartiles, the samples with the lowest levels of E2F5 were most likely to have lymph node metastasis ( P < 0.0001, Fisher test) ( H ).
The ability to model metastases, a feature reflective of human breast cancer, is an attractive component of this new mouse model of breast cancer but one that is offset by the extended latency. To overcome this limitation, we aimed to generate a transplantable metastatic mammary tumor bank. During necropsy of tumor bearing mice, small portions of the tumor were viable frozen. These small tumor fragments were then thawed and directly implanted into mammary glands without passing through tissue culture. MMTV-Cre mice were used as transplant recipients to prevent immune effects associated with the MMTV promoter enhancer 48 . 18 frozen and one fresh tumor were implanted into a small pocket created in the abdominal mammary gland of recipient mice, rapidly resulting in overt tumor formation. Strikingly, it was repeatedly noted that these mice developed a mammary tumor accompanied by a secondary metastatic tumor in the axial lymph node in 12 of the 19 lines at low penetrance. These axial lymph metastases were then implanted into recipient mammary fat pads in repeated rounds of transplantation (Fig. 6A ). The axial lymph node tumors were routinely nearly as large as the primary tumor and an example of the axial lymph node metastasis is shown (top left of the image) after the primary tumor in the abdominal gland was removed at 1 cm endpoint (bottom portion of image) (Fig. 6B ). Tumors only developed where implanted and in the axial lymph nodes. Examining the histology of these metastatic tumors in the axial lymph node revealed the presence of both lymphatic and tumor tissue (Fig. 6C ). Pan-cytokeratin staining for epithelial derived tumor cells revealed nests of tumor cells within this axial lymph node (Fig. 6D ). This was further examined in additional samples where in comparison to the normal lymph node, we were readily able to observe the tumor cells invading the lymph node (Supplemental Figure 15 ). Hypothesizing that the metastasis was occurring through the lymphatic vasculature, we sectioned across the region between the primary tumor and axial lymph node from Fig. 6B and stained for podoplanin, a marker of lymphatic vessel walls. This revealed staining of the vasculature with unstained tumor cells lodged within (Fig. 6E ). Given that blood vasculature does not stain for podoplanin, that the tumor cells were negative for podoplanin and that this mouse developed extensive lymphatic metastasis, the positively stained cells are likely part of the lymphatic vasculature. Control transplants of MMTV-Neu and MMTV-PyMT tumors did not result in lymph node metastasis. To increase the penetrance of lymph node metastasis, we utilized prior enrichment strategies for breast metastases [ 47 , 48 , 49 ] where a sample of lymph node metastases was implanted into the mammary gland of a new recipient mice and the resulting axillary lymph node tumor was re-implanted into new recipient mice. This serial implantation resulted in the enrichment of lymph node metastasis from a penetrance of 23%, 50% and 73% in three separate tumor lines (Fig. 6F ). With each round of implantation, the latency of lymph node metastasis formation was reduced. A summary of the enrichment transplantation revealed the ability across several lines to enrich for this property (Fig. 6F and Supplemental Table 3 ).
After observing lymphatic metastasis in the E2F5 CKO mouse model, we examined the role of E2F5 in human lymphatic metastasis. We predicted E2F5 activity in human breast cancer with known nodal status using the E2F5 signature described in Fig. 1G . The stratification of breast cancers by lymph node metastasis status revealed that those tumors with lymph node metastases had lower levels of E2F5 (Fig. 6G ). Examining the upper and lower quartiles for E2F5 activity revealed almost a four-fold increase in lymphatic metastasis in the lower quartile of E2F5 activity (Fig. 6H ). Together these data indicate that loss of E2F5 is associated with tumors that metastasize to the lymph node in both our conditional knockout mouse model as well as in human breast cancer.
The repressive transcription factor E2F5 has been hypothesized to have a role in mammary gland development and tumor formation. Testing this hypothesis required generation of a conditional deletion due to the early lethality of the traditional E2F5 knockout due to developmental abnormalities [ 19 ]. Surprisingly only minor mammary gland defects were noted with the mammary specific loss of E2F5 but, after an extensive latency, metastatic mammary tumors developed. Notably, these tumors metastasized to the lymph node and this property was enhanced with serial transplantation. Mechanistically, we demonstrated an upregulation of Cyclin D1, with similar expression pattern of Cyclin D family observed in MMTV-Neu, a model where tumorigenicity was dependent upon Cyclin D1 [ 50 ].
During generation of the E2F5 gene signature we overexpressed E2F5 in HMECs and collected RNA 18 h after infection, a standard procedure that allows immediate transcriptional events to be assayed [ 40 , 51 ]. The conventional role for E2F5 is thought to be a transcriptional repressor [ 7 , 32 ], although several more recent publications have demonstrated a role as an activator in diverse settings include zebrafish [ 52 ] and cervical cancer [ 53 ]. Our results demonstrate that E2F5 can function both as an activator and as a repressor in mammary epithelial cells, dependent upon the gene. This is consistent with prior work suggesting that the context of E2F binding is an essential component in determining repressor and activator function [ 54 ].
While the predictive bioinformatic data strongly suggested a role for E2F5 in several stages of mammary development, from regulation of genes expressed in the terminal end bud to stratification of the major stages of mammary development by E2F5 signature genes, the observed phenotype was a relatively minor delay in outgrowth. This was a transient delay with virgin adult mice being indistinguishable from controls and unlike the E2F4 knockouts, the E2F5 CKO mice retained full lactational function of the mammary gland. Prior work has shown a range of individualized roles for E2F1-4 in mammary development with E2F4 having the most severe developmental effects and a near inability to rear pups [ 20 ]. However, consistent with the notion that the E2Fs can compensate for each other [ 21 ], functional compensation during mammary gland development was noted in the E2F knockouts [ 22 ]. The idea of familial compensation was also reinforced by effects seen in E2F4/5 double knockouts that were more extensive than individual knockouts in cell cycle progression. Further, double knockouts suffered from neonatal lethality while the individual knockouts were viable at birth [ 32 ]. Together our data and the literature suggest that E2F5 functions to regulate development in a context dependent manner and other E2Fs may compensate for loss of E2F5 and mitigate the developmental abnormalities.
After an extended latency the E2F5 CKO mice spontaneously developed mammary tumors. Given this latency, we hypothesized that a number of genomic events would be required for transformation and progression to metastatic disease. Integrating our bioinformatic analysis and in vitro studies, we identified a number of potential target genes. While some were unique to individual tumors, including p53 and KRas mutations, other events were shared across the E2F5 conditional knockout tumors. One common event across all tumors was the alteration of Cyclin D1 levels. Cyclin D1 is one of the most commonly amplified and/or overexpressed genes in human breast cancer with overexpression noted in nearly 50% of tumors [ 55 ]. Overexpression of Cyclin D1 in the mouse mammary gland results in mammary tumor development after 18 month latency, supporting a key role in tumorigenesis [ 56 ]. The requirement for Cyclin D1 in various mammary tumor GEMs has previously been demonstrated, including Neu but not Wnt-1. This is largely driven by the effects of other Cyclin family members in the Wnt-1 model while Neu tumors are dependent upon Cyclin D1. Based on gene expression analysis, E2F5 expression is inversely correlated with Cyclin D1, suggesting that E2F5 may be negatively regulating Cyclin D1. In addition, the levels of Cyclin D1, D2 and D3 in E2F5 CKO tumors compared to MMTV-Wnt-1 [ 57 ] and MMTV-Neu [ 58 ] tumors strongly suggest that E2F5 CKO tumors are analogous to MMTV-Neu and are dependent upon Cyclin D1. Indeed, since E2F5 CKO tumors, like MMTV-Neu, mainly expresses Cyclin D1, the E2F5 tumors likely resemble the dependence of MMTV-Neu on Cyclin D1 [ 50 , 59 , 60 ]. Furthermore, Cyclin D1 expression in MMTV-Neu tumors is mediated by E2F1 [ 50 ]. Other studies have also demonstrated that E2F1 and E2F4 can directly bind to and regulate Cyclin D1 expression [ 61 ]. Given the functional redundancy and shared binding motif between E2F family members, it is likely that E2F5 can also regulate Cyclin D1 expression. Taken together, we propose that loss of E2F5 in the mammary gland leads to deregulation of Cyclin D1, contributing to tumor development and progression. Given the wide range of SNV and CNV alterations, we suggest that this occurs in a complex mutational environment with numerous other pathways. Although there is evidence suggesting that E2F5 may be directly Cyclin D1 expression, it is also possible that disruption of E2F5 leads to dysregulation of other targets that can result in Cyclin D1 expression. To confirm the role of Cyclin D1 in E2F5 CKO mice tumorigenesis, our future studies will characterize the effects of loss of Cyclin D1 in the E2F5 CKO model.
The role of Cyclin D1 in comparison to other tumor models also raised the issue of how similar these tumors were to other models. Clearly the tumors were divergent from models such as MMTV-PyMT and MMTV-Neu given the characteristics that included a much longer tumor latency and a propensity for lymphatic metastasis. While a gene expression comparison on tumor data is possible, we have previously shown that these patterns are dominated by the histological subtype [ 44 ]. Instead, we compared the WGS data we previously generated for PyMT and Neu tumors [ 62 ] and compared it with the E2F5 CKO tumors, which revealed a unique mutational spectrum. Despite this, the E2F5 CKO tumors share the Cyclin family pattern with Neu tumors. This suggests some similarity to the Neu tumors but the mutational analysis also provides key differences.
In the study of mammary tumors that spontaneously metastasize in genetically engineered mouse models, pulmonary metastasis is typically noted, a feature that was also observed in the E2F5 CKO mice. Interestingly, we discovered that E2F5 CKO mammary tumors transplanted into the abdominal mammary fat pad had a propensity to metastasize to the axillary lymph node. Given that axillary lymph nodes are most commonly the first site of metastasis in human breast cancer, we sought to enrich the ability of E2F5 CKO tumors to metastasize to the axillary lymph node. Using a serial transplantation technique to re-transplant the axillary tumor into the abdominal mammary fat pad, we generated a syngeneic transplantation model that developed lymph node metastasis within one month of transplant and with >80% penetrance. Generally, the current mouse models of breast cancer rarely metastasize to the lymph node [ 63 ]. Thus, this model of enriched lymph node tumors is unique and can be a tool to examine the mechanisms driving lymph node metastasis. However, it should be noted that this transplantation technique includes a 1 x 1x 1 mm fragment of the tumor which includes cells from the microenvironment, including other cell types such as fibroblasts, endothelial cells and a variety of immune cells and this may alter the properties and signaling of the cancer cells.
Taken together, we have identified a novel role of E2F5 as a function tumor suppressor utilizing a conditional knockout mouse model. This is consistent with prior literature suggesting E2F5 has varied roles, including as a tumor suppressor [ 33 ]. In contrast, there are studies suggesting E2F5 may behave as an oncogenes in various cancer types including breast cancer. For example, a previous study demonstrates that knocking down E2F5 in human breast cancer cell lines inhibited cell proliferation, migration and invasion [ 64 ]. It is unclear why there is conflicting data on the role of E2F5 as an oncogene or tumor suppressor but, as with many genes, context is key. Importantly, all previous studies describing the oncogenic role of E2F5 in breast cancer have been completed in cell line models, whereas this is the first study that characterizes loss of E2F5 in the mammary gland of a mouse model. Interestingly, a similar paradigm exists for E2F8, an atypical E2F family member, where a possible oncogenic role was uncovered when knocked down in transformed cells and a mouse model knockout revealed a tumor suppressor effect [ 65 , 66 , 67 , 68 ]. We theorize that like E2F8 and other E2F family members, E2F5 may behave as an oncogene or tumor suppressor depending on context. Importantly, this context may be dependent upon tumor type, and on when the dysregulation of E2F5 occurs, during precancerous vs transformed stage.
This study has resulted in the development of a novel mouse model of breast cancer with histologically diverse mammary tumors and metastatic lesions. A unique feature of this model is its prolonged tumor latency which is similar to human breast cancers, where the majority of cancers occur in older postmenopausal women. Although breast cancer is an age-related disease, the role of aging in tumorigenesis has not been well defined. Studying age-related impacts on tumorigenesis has been limited in part by the availability of transgenic mouse models with prolonged tumor latency [ 69 ]. Since the majority of available transgenic mouse models develop tumors rapidly, it does not allow time for age-related changes to occur. Thus, E2F5CKO mice, which has an average tumor latency of 18–21 months, may be a favorable model to elucidate the role of aging in breast cancer development and progression. Finally, the E2F5 CKO syngeneic transplantation model can be a significant resource to studying the mechanism of lymphatic metastasis.
Material and methods
E2f5 expression in mammary development stages and cell types.
To analyze E2F5 expression in a single cell RNA-seq mammary development dataset, the following website was used: https://marionilab.cruk.cam.ac.uk/mammaryGland/ . To analyze E2F5 expression in various mammary cell types, the following website was used: https://bis.zju.edu.cn/MCA/ .
Mouse dataset usage
The following published microarray datasets were used for analysis: terminal end bud and duct (GSE2988) and mammary gland developmental stages (GSE12247).
Newly generated RNAseq and WGS data has been deposited at the NIH NLM under BioProject # PRJNA887715.
E2F5 regulated genes
Human Mammary Epithelial cells were infected with adenovirus expressing E2F5 or GFP. Cells were collected eighteen hours after infection. Total RNA was extracted using Qiagen RNeasy Mini kit and submitted to Michigan State University Genomics Core facility for gene expression analysis using Affymetrix Human Genome U133 chip. Robust Multichip Average (RMA) algorithm was used to normalized microarray dataset. Significance Analysis of Microarray was used to identify differentially expressed genes in HMEC-E2F5.
E2F5 activation signature generation
E2F5 activation signature was generated based on previously described bayesian approach [ 40 ]. The E2F5 activation signature was applied to publicly available human breast cancer cell line data set GSE3156. To validate the signature, E2F5 expression was evaluated in two cell lines with the highest predicted E2F5 activity and two cell lines with the lowest predicted E2F5 activity via Western blot.
Cluster 3.0 was used to perform unsupervised hierarchical clustering. Heatmap was generated using MATLAB imagesc function and Broad institute’s Morpheus and Genepattern [ 70 ] interface.
Gene ontology
The subset of E2F5 regulated genes enriched in each mammary developmental stage were characterized by gene ontology using https://maayanlab.cloud/Enrichr/ (Kuleshov et al. 2016).
Animal generation
All animal husbandry and use was in compliance with local, national and institutional guidelines. Ethical approval for the study was approved by Michigan State University Animal Care & Use Committee (IACUC) under AUF 06/18-084-00. E2F5 CKO mice were generated by flanking exons 2 and 3 of the E2F5 gene with loxP sites. E2F5 flox/flox mice were interbred with MMTV-Cre mice [ 42 ]. Mice were monitored weekly for tumor development. The endpoint for primary tumor was 2000 mm 3 .
For wholemount analysis, abdominal mammary fat pads were excised and placed on glass slides. The slides were incubated in acetone for 24 h, rehydrated through an ethanol progression and stained in Harris’ Modified Hematoxylin for 24 h. The slides were destained in acidified ethanol. Slides were dehydrated and mounted with permount. To evaluate mammary outgrowth, the distance from the nipple to the leading edge of the epithelium and the distance from the nipple to the midpoint of the thoracic lymph node were measured. Samples for histology were fixed in 10% formalin and submitted to Michigan State University Pathology lab.
Whole genome sequence generation and analysis
Whole genome sequencing (WGS) sample reads were concatenated and quality checked using FastQC/0.11.7. Sample reads had adapter ends trimmed off using Trimmomatic/0.38 [ 71 ] and again checked for quality using FastQC. Paired WGS reads were aligned to the GRCm38-mm10 reference genome using Burrows-Wheeler Aligner/0.7.17 [ 72 ]. Read groups were added using Picard/2.22.1 from the Broad Institute http://broadinstitute.github.io/picard . Reads were then indexed and sorted using SAMtools/1.11 [ 73 ]. Duplicate reads were removed using Picard.
Variant calling
Final bam files had single nucleotide variants (SNVs) called using the consensus of Mutect2 under GATK/4.0.5.1 [ 74 ] and VarScan/2.4.1 [ 75 ]. Translocations, inversions, and copy number variants (CNVs) were called using the consensus of Delly/0.7.8 [ 76 ] and Lumpy/0.2.13 [ 77 ] for each tumor sample. Annotations of all samples were done using SnpEff/4.1d [ 78 ]. Tabular data from tumor VCF files were sorted and processed using a custom Python script using Python 3.8.8, Pandas 1.2.4, and NumPy 1.20.1. All unaltered VCF files are available in supplementary.
Circos visualization
Circos plots were generated using Circos/0.69-6 [ 79 ].
CNVkit Plots
CNVkit plots were generated on CNVKit/0.9.9 [ 80 ]. The “cnvkit.py batch –method wgs” option was used to generate the copy number ratios and copy segments files for downstream visualization. The “cnvkit.py scatter” command was used to generate the scatter plots with all default setting maintained. Heatmaps were generated using the “cnvkit.py heatmap -d” options. Tumor calls were made against normal FVB tissue (ERR046395). To reduce false positives, we ran our same pipeline on the Sanger Mouse Genomes Project ( https://www.sanger.ac.uk/data/mouse-genomes-project/ ) FVB/NJ background release 1604 bam file. All SNVs found in either FVB background were filtered out from the tumor data. Only SNVs with a somatic p -value <= 0.05 were included in all downstream analyses as determined by VarScan. CNVs and inversions found in the FVB/NJ background that are on the same chromosome and have a difference of less than 100 bps in length from either FVB background event are excluded from analysis. For translocations, all events on either FVB background that are within 1 kb of either the donor or receiver position on each chromosome are dropped. These steps were accomplished using a custom python script.
Mutation signature
The Catalogue of Somatic Mutations in Cancer (COSMIC) single base substitution (SBS) signatures for E2F5 flox/flox tumors were derived using the deconstructSigs R package [ 81 ]. The mouse GRCm38 (mm10) reference assembly was used for trinucleotide context when calling SBS signatures. The resulting weights were plotted using matplotlib in Python.
Cell culture
Human mammary epithelial cells were cultured in Mammary Epithelial Cell Basal Medium (ATCC PCS-600-030) supplemented with rH-insulin (5 ug/mL), L-glutamine (6 mM), epinephrine (1 µM), apo-transferrin (5 µg/ml), rH-TGF-α (5 ng/ml), extractP (0.4%) and hydrocortisone hemisuccinate (100 ng/ml).
E2F5KO tumor cell line generation
E2F5KO mammary tumor pieces were placed on a culture dish with Dulbecco’s Modified Eagle Medium (Millipore Sigma D5030) supplemented with 10% Fetal bovine serum and 1x Antibiotic/Antimycotic to allow for cells to dissociate. Cells were passaged several times to enrich for epithelial cell population. Western blot was using an E2F5 antibody to demonstrate loss of E2F5 in isolated cell lines (Supplemental Fig. 16 ). Tumor cell lines were validated for tumorigenic potential by implantation into recipient (MMTV-Cre) lines where they were noted to form tumors.
RNA-sequencing
Flash frozen tumor pieces were homogenized using Fisher Homogenizer 150 (Thermo Scientific). Total RNA was isolated using QIAGEN RNeasy Midi Kit (Hilden, Germany #75142) with the manufacturer’s protocol. RNA concentration was measured by Qubit (Thermo Scientific) and Agilent 2100 Bioanalyzer. RNA was submitted to Novogene to generate gene expression data. RNA samples with RIN > 7 was used for library preparation using the Illumina Tru-Seq stranded total RNA kit. RNA library was sequenced to a depth of >20 M reads/sample with paired end 150 base paired reads on Illumina NovaSeq 6000. Adaptors were removed from reads using Trimmomatic v0.33. Quality control was performed using FastQC v0.11.5. Reads were aligned and mapped using STAR [ 82 ]. RSEM was used to quantify and normalize reads [ 83 ]. Differential gene expression analysis was performed using EdgeR [ 84 ]. Pathway analysis was performed using https://maayanlab.cloud/Enrichr/ [ 46 ]. Protein-protein interaction network was generated with https://string-db.org/ [ 85 ].
Quantitative RT-PCR
Flash frozen tumor pieces were homogenized using Fisher Homogenizer 150 (Thermo Fisher). Total RNA was isolated using QIAGEN RNeasy Midi Kit (Qiagen 75142) with the manufacturer’s protocol. Quantitative RT-PCR was performed using Luna Universal One-Step RT-qPCR kit (New England Biolabs E3005S) according to manufacturer’s protocol using Agilent Mx3000P instrument. Primers were designed using Primer Bank tool ( https://pga.mgh.harvard.edu/primerbank/ ). The following primers were used (5’ to 3’): CCND1 forward, TGACTGCCGAGAAGTTGTGC ; CCND1 reverse, CTCATCCGCCTCTGGCATT ; Gapdh forward, AGGTCGGTGTGAACGGATTTG ; Gapdh reverse, TGTAGACCATGTAGTTGAGGTCA . Primer efficiency was 90–110% for all primers used. Delta-delta CT method was used for fold change analysis. For mammary gland comparisons, 3 control and 3 conditional knockout samples were used and experiments were done in triplicate.
Immunoblotting
To extract RNA from tissue, samples were homogenized using mortar and pestle in liquid nitrogen. Sample were lysed in RIPA lysis buffer (Thermo Scientific 89900) with proteinase inhibitor (Thermo Scientific A32963) for 1 h on ice with constant agitation. Protein was quantitated using BCA Protein Assay Kit (Thermo Scientific 3225) and then boiled at 100 °C for 5 min. Samples were loaded onto an 8–12% polyacrylamide gel. Separated protein was transferred onto an Immobilon- FL PVDF membrane (Millipore Sigma PFL00010). Membranes were blocked in 5% milk in TBS with 0.1% Tween-20 (TBS-Tween) for 1 h and then incubated in primary antibody overnight at 4 °C. Following three washes in TBS-Tween the membrane was incubated in the appropriate antibody at a dilution 1:10,000 in 5% milk in TBS-Tween for 1 h at room temperature. Membranes were washed 3x in TBS-Tween and imaged on LI-COR Odyssey imaging system. The following antibodies were used: 1:100 E2F5 (Santa Cruz Biotech sc-374268), 1:1000 Grb2 (Cell Signaling Technology 3976), 1:4000 Vinculin (Cell Signaling Technology 13901), 1:2000 Cyclin D1 (Thermo Scientific 516356.), 1:2000 Cyclin D3 (Thermo Scientific PA5-97551) and 1:1000 Cyclin D2 (Proteintech 10934-1-AP). Image studio version 5.5 was used for analysis and quantification of the Western blot.
Mammary fat pad transplantation
E2F5 CKO mammary tumors were harvested and stored in DMEM with 20% FBS and 10% DMSO at −80 °C before long term storage in liquid nitrogen vapor phase. Tumors were thawed and orthotopically implanted into the abdominal mammary gland of 6-to-10-week old MMTV Cre female mice. Mice were palpated 2x a week for mammary tumor formation. When the tumor size reached 2000 mm 3 , samples were harvested for further analysis.
E2F5 activation in clinical samples
E2F5 activation signature was applied to a human breast cancer database using MATLAB [ 86 ]. Graphpad was used to create the survival plot in patients with high vs low E2F5 activation. Broad Institute’s Morpheus was used to generate the heatmap demonstrating E2F5 activation in human breast cancer patient and its lymph node metastasis status.
Statistical analysis
Except otherwise noted, all statistical comparisons are performed with an unpaired students two-tailed, unpaired t -test.
Data availability
Data is freely available and bioinformatic data has been deposited at BioProject # PRJNA887715.
Stein T, Morris JS, Davies CR, Weber-Hall SJ, Duffy MA, Heath VJ, et al. Involution of the mouse mammary gland is associated with an immune cascade and an acute-phase response, involving LBP, CD14 and STAT3. Breast Cancer Res. 2004;6:R75–91.
Article CAS PubMed Google Scholar
Clarkson RW, Wayland MT, Lee J, Freeman T, Watson CJ. Gene expression profiling of mammary gland development reveals putative roles for death receptors and immune mediators in post-lactational regression. Breast Cancer Res. 2004;6:R92–109.
Dyson N. The regulation of E2F by pRB-family proteins. Genes Dev. 1998;12:2245–62.
Van Den Heuvel S, Dyson NJ. Conserved functions of the pRB and E2F families. Nature reviews . Mol cell Biol. 2008;9:713–24.
Google Scholar
Trimarchi JM, Lees JA. Sibling rivalry in the E2F family. Nat Rev Mol Cell Biol. 2002;3:11–20.
Sears RC, Nevins JR. Signaling networks that link cell proliferation and cell fate. J Biol Chem. 2002;277:11617–20.
Attwooll C, Lazzerini Denchi E, Helin K. The E2F family: specific functions and overlapping interests. EMBO J. 2004;23:4709–16.
Article CAS PubMed PubMed Central Google Scholar
Johnson DG, Schwarz JK, Cress WD, Nevins JR. Expression of transcription factor E2F1 induces quiescent cells to enter S phase. Nature. 1993;365:349–52.
Laresgoiti U, Apraiz A, Olea M, Mitxelena J, Osinalde N, Rodriguez JA, et al. E2F2 and CREB cooperatively regulate transcriptional activity of cell cycle genes. Nucleic Acids Res. 2013;41:10185–98.
Mudryj M, Devoto SH, Hiebert SW, Hunter T, Pines J, Nevins JR. Cell cycle regulation of the E2F transcription factor involves an interaction with cyclin A. Cell. 1991;65:1243–53.
Hallstrom TC, Mori S, Nevins JR. An E2F1-dependent gene expression program that determines the balance between proliferation and cell death. Cancer Cell. 2008;13:11–22.
Asp P, Acosta-Alvear D, Tsikitis M, van Oevelen C, Dynlacht BD. E2f3b plays an essential role in myogenic differentiation through isoform-specific gene regulation. Genes Dev. 2009;23:37–53.
Chong JL, Wenzel PL, Saenz-Robles MT, Nair V, Ferrey A, Hagan JP, et al. E2f1-3 switch from activators in progenitor cells to repressors in differentiating cells. Nature. 2009;462:930–4.
Fajas L. Metabolic control in cancer cells. Ann Endocrinol (Paris). 2013;74:71–73.
Field SJ, Tsai FY, Kuo F, Zubiaga AM, Kaelin WG Jr., Livingston DM, et al. E2F-1 functions in mice to promote apoptosis and suppress proliferation. Cell. 1996;85:549–61.
Murga M, Fernandez-Capetillo O, Field SJ, Moreno B, Borlado LR, Fujiwara Y, et al. Mutation of E2F2 in mice causes enhanced T lymphocyte proliferation, leading to the development of autoimmunity. Immunity. 2001;15:959–70.
Humbert PO, Verona R, Trimarchi JM, Rogers C, Dandapani S, Lees JA. E2f3 is critical for normal cellular proliferation. Genes Dev. 2000;14:690–703.
Rempel RE, Saenz-Robles MT, Storms R, Morham S, Ishida S, Engel A, et al. Loss of E2F4 activity leads to abnormal development of multiple cellular lineages. Mol Cell. 2000;6:293–306.
Lindeman GJ, Dagnino L, Gaubatz S, Xu Y, Bronson RT, Warren HB, et al. A specific, nonproliferative role for E2F-5 in choroid plexus function revealed by gene targeting. Genes Dev. 1998;12:1092–8.
Andrechek ER, Mori S, Rempel RE, Chang JT, Nevins JR. Patterns of cell signaling pathway activation that characterize mammary development. Development. 2008;135:2403–13.
Kong LJ, Chang JT, Bild AH, Nevins JR. Compensation and specificity of function within the E2F family. Oncogene. 2007;26:321–7.
To B, Andrechek ER. Transcription factor compensation during mammary gland development in E2F knockout mice. PLoS One. 2018;13:e0194937.
Article PubMed PubMed Central Google Scholar
Fujiwara K, Yuwanita I, Hollern DP, Andrechek ER. Prediction and Genetic Demonstration of a Role for Activator E2Fs in Myc-Induced Tumors. Cancer Res. 2011;71:1924–32.
Yuwanita I, Barnes D. S, Andrechek ER. Increased metastasis with loss of E2F2 in Myc-driven tumors. Oncotarget. 2015;6:38210–24.
Hollern DP, Honeysett J, Cardiff RD, Andrechek ER. The E2F transcription factors regulate tumor development and metastasis in a mouse model of metastatic breast cancer. Mol Cell Biol. 2014;34:3229–43.
Andrechek ER. HER2/Neu tumorigenesis and metastasis is regulated by E2F activator transcription factors. Oncogene. 2015;34:217–25.
Rennhack J, Andrechek E. Conserved E2F mediated metastasis in mouse models of breast cancer and HER2 positive patients. Oncoscience. 2015;2:867–71.
Black EP, Hallstrom T, Dressman HK, West M, Nevins JR. Distinctions in the specificity of E2F function revealed by gene expression signatures. Proc Natl Acad Sci USA. 2005;102:15948–53.
Leone G, Sears R, Huang E, Rempel R, Nuckolls F, Park CH, et al. Myc requires distinct E2F activities to induce S phase and apoptosis. Mol Cell. 2001;8:105–13.
Pusapati RV, Weaks RL, Rounbehler RJ, McArthur MJ, Johnson DG. E2F2 suppresses Myc-induced proliferation and tumorigenesis. Mol Carcinog. 2010;49:152–6.
Rounbehler RJ, Rogers PM, Conti CJ, Johnson DG. Inactivation of E2f1 enhances tumorigenesis in a Myc transgenic model. Cancer Res. 2002;62:3276–81.
CAS PubMed Google Scholar
Gaubatz S, Lindeman GJ, Ishida S, Jakoi L, Nevins JR, Livingston DM, et al. E2F4 and E2F5 play an essential role in pocket protein-mediated G1 control. Mol Cell. 2000;6:729–35.
Pierce AM, Schneider-Broussard R, Philhower JL, Johnson DG. Differential activities of E2F family members: unique functions in regulating transcription. Mol Carcinog. 1998;22:190–8.
Chen C, Wells AD. Comparative analysis of E2F family member oncogenic activity. PLoS One. 2007;2:e912.
Jiang Y, Yim SH, Xu HD, Jung SH, Yang SY, Hu HJ, et al. A potential oncogenic role of the commonly observed E2F5 overexpression in hepatocellular carcinoma. World J Gastroenterol. 2011;17:470–7.
Polanowska J, Le Cam L, Orsetti B, Valles H, Fabbrizio E, Fajas L, et al. Human E2F5 gene is oncogenic in primary rodent cells and is amplified in human breast tumors. Genes Chromosomes Cancer. 2000;28:126–30.
Umemura S, Shirane M, Takekoshi S, Kusakabe T, Itoh J, Egashira N, et al. Overexpression of E2F-5 correlates with a pathological basal phenotype and a worse clinical outcome. Br J Cancer. 2009;100:764–71.
Bach K, Pensa S, Grzelak M, Hadfield J, Adams DJ, Marioni JC, et al. Differentiation dynamics of mammary epithelial cells revealed by single-cell RNA sequencing. Nat Commun. 2017;8:2128.
Han X, Wang R, Zhou Y, Fei L, Sun H, Lai S, et al. Mapping the Mouse Cell Atlas by Microwell-Seq. Cell. 2018;173:1307.
Bild AH, Yao G, Chang JT, Wang Q, Potti A, Chasse D, et al. Oncogenic pathway signatures in human cancers as a guide to targeted therapies. Nature. 2006;439:353–7.
Kouros-Mehr H, Werb Z. Candidate regulators of mammary branching morphogenesis identified by genome-wide transcript analysis. Dev Dyn. 2006;235:3404–12.
Andrechek ER, Hardy WR, Siegel PM, Rudnicki MA, Cardiff RD, Muller WJ. Amplification of the neu/erbB-2 oncogene in a mouse model of mammary tumorigenesis. Proc Natl Acad Sci USA. 2000;97:3444–9.
Andrechek ER, White D, Muller WJ. Targeted disruption of ErbB2/Neu in the mammary epithelium results in impaired ductal outgrowth. Oncogene. 2005;24:932–7.
Hollern DP, Swiatnicki MR, Andrechek ER. Histological subtypes of mouse mammary tumors reveal conserved relationships to human cancers. PLoS Genet. 2018;14:e1007135.
Gyorffy B. Survival analysis across the entire transcriptome identifies biomarkers with the highest prognostic power in breast cancer. Comput Struct Biotechnol J. 2021;19:4101–9.
Kuleshov MV, Jones MR, Rouillard AD, Fernandez NF, Duan Q, Wang Z, et al. Enrichr: a comprehensive gene set enrichment analysis web server 2016 update. Nucleic Acids Res. 2016;44:W90–97.
Bos PD, Zhang XH, Nadal C, Shu W, Gomis RR, Nguyen DX, et al. Genes that mediate breast cancer metastasis to the brain. Nature. 2009;459:1005–9.
Kang Y, Siegel PM, Shu W, Drobnjak M, Kakonen SM, Cordon-Cardo C, et al. A multigenic program mediating breast cancer metastasis to bone. Cancer Cell. 2003;3:537–49.
Minn AJ, Gupta GP, Siegel PM, Bos PD, Shu W, Giri DD, et al. Genes that mediate breast cancer metastasis to lung. Nature. 2005;436:518–24.
Lee RJ, Albanese C, Fu M, D’Amico M, Lin B, Watanabe G, et al. Cyclin D1 is required for transformation by activated Neu and is induced through an E2F-dependent signaling pathway. Mol Cell Biol. 2000;20:672–83.
Gatza ML, Lucas JE, Barry WT, Kim JW, Wang Q, Crawford MD, et al. A pathway-based classification of human breast cancer. Proc Natl Acad Sci USA. 2010;107:6994–9.
Xie H, Kang Y, Wang S, Zheng P, Chen Z, Roy S, et al. E2f5 is a versatile transcriptional activator required for spermatogenesis and multiciliated cell differentiation in zebrafish. PLoS Genet. 2020;16:e1008655.
Teissier S, Pang CL, Thierry F. The E2F5 repressor is an activator of E6/E7 transcription and of the S-phase entry in HPV18-associated cells. Oncogene. 2010;29:5061–70.
Freedman JA, Chang JT, Jakoi L, Nevins JR. A combinatorial mechanism for determining the specificity of E2F activation and repression. Oncogene. 2009;28:2873–81.
Buckley MF, Sweeney KJ, Hamilton JA, Sini RL, Manning DL, Nicholson RI, et al. Expression and amplification of cyclin genes in human breast cancer. Oncogene. 1993;8:2127–33.
Wang TC, Cardiff RD, Zukerberg L, Lees E, Arnold A, Schmidt EV. Mammary hyperplasia and carcinoma in MMTV-cyclin D1 transgenic mice. Nature. 1994;369:669–71.
Li Y, Hively WP, Varmus HE. Use of MMTV-Wnt-1 transgenic mice for studying the genetic basis of breast cancer. Oncogene. 2000;19:1002–9.
Guy CT, Webster MA, Schaller M, Parsons TJ, Cardiff RD, Muller WJ. Expression of the neu protooncogene in the mammary epithelium of transgenic mice induces metastatic disease. Proc Natl Acad Sci USA. 1992;89:10578–82.
Yu Q, Geng Y, Sicinski P. Specific protection against breast cancers by cyclin D1 ablation. Nature. 2001;411:1017–21.
Zhang Q, Sakamoto K, Liu C, Triplett AA, Lin WC, Rui H, et al. Cyclin D3 compensates for the loss of cyclin D1 during ErbB2-induced mammary tumor initiation and progression. Cancer Res. 2011;71:7513–24.
Watanabe G, Albanese C, Lee RJ, Reutens A, Vairo G, Henglein B, et al. Inhibition of cyclin D1 kinase activity is associated with E2F-mediated inhibition of cyclin D1 promoter activity through E2F and Sp1. Mol Cell Biol. 1998;18:3212–22.
Rennhack JP, To B, Swiatnicki M, Dulak C, Ogrodzinski MP, Zhang Y, et al. Integrated analyses of murine breast cancer models reveal critical parallels with human disease. Nat Commun. 2019;10:3261.
To B, Isaac D, Andrechek ER. Studying Lymphatic Metastasis in Breast Cancer: Current Models, Strategies, and Clinical Perspectives. J Mammary Gland Biol Neoplasia. 2020;25:191–203.
Article PubMed Google Scholar
Xu H, Fei D, Zong S, Fan Z. MicroRNA-154 inhibits growth and invasion of breast cancer cells through targeting E2F5. Am J Transl Res. 2016;8:2620–30.
CAS PubMed PubMed Central Google Scholar
Thurlings I, Martinez-Lopez LM, Westendorp B, Zijp M, Kuiper R, Tooten P, et al. Synergistic functions of E2F7 and E2F8 are critical to suppress stress-induced skin cancer. Oncogene. 2017;36:829–39.
Kent LN, Rakijas JB, Pandit SK, Westendorp B, Chen HZ, Huntington JT, et al. E2f8 mediates tumor suppression in postnatal liver development. J Clin Invest. 2016;126:2955–69.
Park SA, Platt J, Lee JW, López-Giráldez F, Herbst RS, Koo JS. E2F8 as a Novel Therapeutic Target for Lung Cancer. J Natl Cancer Inst. 2015;107. https://doi.org/10.1093/jnci/djv151 .
Deng Q, Wang Q, Zong WY, Zheng DL, Wen YX, Wang KS, et al. E2F8 contributes to human hepatocellular carcinoma via regulating cell proliferation. Cancer Res. 2010;70:782–91.
Rennhack J, To B, Wermuth H, Andrechek ER. Mouse Models of Breast Cancer Share Amplification and Deletion Events with Human Breast Cancer. J Mammary Gland Biol Neoplasia. 2017;22:71–84.
Reich M, Liefeld T, Gould J, Lerner J, Tamayo P, Mesirov JP. GenePattern 2.0. Nat Genet. 2006;38:500–1.
Bolger AM, Lohse M, Usadel B. Trimmomatic: a flexible trimmer for Illumina sequence data. Bioinformatics. 2014;30:2114–20.
Li H, Durbin R. Fast and accurate short read alignment with Burrows-Wheeler transform. Bioinformatics. 2009;25:1754–60.
Li H, Handsaker B, Wysoker A, Fennell T, Ruan J, Homer N, et al. The Sequence Alignment/Map format and SAMtools. Bioinformatics. 2009;25:2078–9.
McKenna A, Hanna M, Banks E, Sivachenko A, Cibulskis K, Kernytsky A, et al. The Genome Analysis Toolkit: a MapReduce framework for analyzing next-generation DNA sequencing data. Genome Res. 2010;20:1297–303.
Koboldt DC, Zhang Q, Larson DE, Shen D, McLellan MD, Lin L, et al. VarScan 2: somatic mutation and copy number alteration discovery in cancer by exome sequencing. Genome Res. 2012;22:568–76.
Rausch T, Zichner T, Schlattl A, Stutz AM, Benes V, Korbel JO. DELLY: structural variant discovery by integrated paired-end and split-read analysis. Bioinformatics. 2012;28:i333–i339.
Layer RM, Chiang C, Quinlan AR, Hall IM. LUMPY: a probabilistic framework for structural variant discovery. Genome Biol. 2014;15:R84.
Cingolani P, Platts A, Wang le L, Coon M, Nguyen T, Wang L, et al. A program for annotating and predicting the effects of single nucleotide polymorphisms, SnpEff: SNPs in the genome of Drosophila melanogaster strain w1118; iso-2; iso-3. Fly (Austin). 2012;6:80–92.
Krzywinski M, Schein J, Birol I, Connors J, Gascoyne R, Horsman D, et al. Circos: an information aesthetic for comparative genomics. Genome Res. 2009;19:1639–45.
Talevich E, Shain AH, Botton T, Bastian BC. CNVkit: Genome-Wide Copy Number Detection and Visualization from Targeted DNA Sequencing. PLoS Comput Biol. 2016;12:e1004873.
Rosenthal R, McGranahan N, Herrero J, Taylor BS, Swanton C. DeconstructSigs: delineating mutational processes in single tumors distinguishes DNA repair deficiencies and patterns of carcinoma evolution. Genome Biol. 2016;17:31.
Dobin A, Davis CA, Schlesinger F, Drenkow J, Zaleski C, Jha S, et al. STAR: ultrafast universal RNA-seq aligner. Bioinformatics. 2013;29:15–21.
Li B, Dewey CN. RSEM: accurate transcript quantification from RNA-Seq data with or without a reference genome. BMC Bioinforma. 2011;12:323.
Article CAS Google Scholar
Robinson MD, McCarthy DJ, Smyth GK. edgeR: a Bioconductor package for differential expression analysis of digital gene expression data. Bioinformatics. 2010;26:139–40.
von Mering C, Jensen LJ, Snel B, Hooper SD, Krupp M, Foglierini M, et al. STRING: known and predicted protein-protein associations, integrated and transferred across organisms. Nucleic Acids Res. 2005;33:D433–437.
Article Google Scholar
Mihaly Z, Kormos M, Lanczky A, Dank M, Budczies J, Szasz MA, et al. A meta-analysis of gene expression-based biomarkers predicting outcome after tamoxifen treatment in breast cancer. Breast Cancer Res Treat. 2013;140:219–32.
Download references
This work was supported by a grant from the Elsa U Pardee Foundation and NICHD 1R01HD104606-01 to ERA, and Aitch Foundation scholarships to BT and JPR.
Author information
Deceased: David Judah.
Authors and Affiliations
Department of Physiology, Michigan State University, East Lansing, MI, USA
Briana To, Carson Broeker, Jing-Ru Jhan, Jesus Garcia-Lerena, John Vusich, Jonathan P. Rennhack, Lauren Jackson, David Judah, Matt Swiatnicki, Evan Bylett, Rachel Kubiak, Jordan Honeysett & Eran Andrechek
Duke University, Durham, USA
Rachel Rempel & Joseph Nevins
The Salk Institute, San Diego, CA, USA
Daniel Hollern
You can also search for this author in PubMed Google Scholar
Contributions
BT was involved with conceptualization, methodology, investigation, and writing, CB analyzed data, J-RJ was involved in methodology and investigation, JG-L, JV, RR, JR, DH, LJ, DJ, MS, EV, RK and JH were involved in analyzing data and investigation, JN was involved in conceptualization and ERA was involved in conceptualization, methodology, validation, analysis, investigation, writing, supervision, administration and funding acquisition.
Corresponding author
Correspondence to Eran Andrechek .
Ethics declarations
Competing interests.
The authors declare no competing interests.
Additional information
Publisher’s note Springer Nature remains neutral with regard to jurisdictional claims in published maps and institutional affiliations.
Supplementary information
Supplemental material, supplemental table 1, supplemental table 2, supplemental table 3, supplemental table 4, rights and permissions.
Open Access This article is licensed under a Creative Commons Attribution-NonCommercial-NoDerivatives 4.0 International License, which permits any non-commercial use, sharing, distribution and reproduction in any medium or format, as long as you give appropriate credit to the original author(s) and the source, provide a link to the Creative Commons licence, and indicate if you modified the licensed material. You do not have permission under this licence to share adapted material derived from this article or parts of it. The images or other third party material in this article are included in the article’s Creative Commons licence, unless indicated otherwise in a credit line to the material. If material is not included in the article’s Creative Commons licence and your intended use is not permitted by statutory regulation or exceeds the permitted use, you will need to obtain permission directly from the copyright holder. To view a copy of this licence, visit http://creativecommons.org/licenses/by-nc-nd/4.0/ .
Reprints and permissions
About this article
Cite this article.
To, B., Broeker, C., Jhan, JR. et al. Insight into mammary gland development and tumor progression in an E2F5 conditional knockout mouse model. Oncogene (2024). https://doi.org/10.1038/s41388-024-03172-4
Download citation
Received : 12 October 2023
Revised : 23 August 2024
Accepted : 16 September 2024
Published : 28 September 2024
DOI : https://doi.org/10.1038/s41388-024-03172-4
Share this article
Anyone you share the following link with will be able to read this content:
Sorry, a shareable link is not currently available for this article.
Provided by the Springer Nature SharedIt content-sharing initiative
Quick links
- Explore articles by subject
- Guide to authors
- Editorial policies


IMAGES
VIDEO
COMMENTS
Experimental mouse tumour models have provided key mechanistic insights into host antitumour immune responses, and these have guided the development of novel treatment strategies. To accelerate ...
Although syngeneic mouse models using 4T1 and E0771 mouse mammary tumour cells 47 and colorectal tumours transplanted onto the colonic mucosa 48 have been established and demonstrated to undergo ...
2. Animal models for spontaneous tumors. Spontaneous animal tumor models are utilized to investigate tumors that occur naturally in experimental animals without any conscious artificial intervention, and this occurrence type and incidence vary greatly with different species and strains of experimental animal (Figure 1).The advantage of the spontaneous animal tumor model is that the process of ...
Microsurgical techniques are helpful to obtain experimental cancer models especially because of their availability, as well as their rapid and visible results. ... Sundberg J.P., Eppig J.T. Electronic access to data from mouse cancer models: The Mouse Tumor Biology database. Nat. Genet. 2001; 27:65-66. doi: 10.1038/87160. [Google Scholar]
Preclinical mouse tumour models therefore serve as an indispensable intermediate experimental model system bridging more reductionist in vitro research with human studies.
Experimental mouse tumour models have provided key mechanistic insights into host antitumour immune responses, and these have guided the development of novel treatment strategies. To accelerate the translation of these findings into clinical benefits, investigators need to gain a better understanding of the strengths and limitations of mouse ...
This attrition can be attributed, at least in part, to the clinical development being underpinned by the demonstration of predictable efficacy in experimental models of human tumours. This review will focus on the range of models available for the discovery and development of anticancer drugs, from traditional subcutaneous injection of tumour ...
The interpretation of experimental results in mouse models can also be confounded when researchers do not factor in the effect of genetic background on tumor biology. The Mouse Tumor Biology (MTB) database is an expertly curated, comprehensive compendium of mouse models of human cancer. Through the enforcement of nomenclature and related ...
Experimental mouse tumour models have provided key mechanistic insights into host antitumour immune responses, and these have guided the development of novel treatment strategies. To accelerate the translation of these findings into clinical benefits, investigators need to gain a better understanding of the strengths and limitations of mouse ...
PDX Mouse Models Are Reliable Stand-Ins for Human Tumors, Study Finds. PDX mice largely retain the genetics of the human tumors from which they were initially created, a new study shows. Credit: Adapted from image provided courtesy of Jackson Laboratory. Mouse models are among the most valuable tools in cancer research.
various tumour cell lines, and preferably also other experimental cancer models. 5.4.1 Xenograft Models The term xenograft refers to the transplantation of cultured human tumour cells or human tumour explants into immunodeficient mice which do not reject the graft. The most common immunodeficient mouse strains used in xenograft models are
Extensive research by both the academic and pharmaceutical communities over the past 30 years has greatly increased the range of mouse tumour models available. This review will focus on (i) the range of models available during the discovery and development of anticancer drugs, (ii) how these models add value to the drug discovery process and ...
Experimental mouse tumour models have provided key mechanistic insights into host antitumour immune responses, and these have guided the development of novel treatment strategies, but investigators need to gain a better understanding of their strengths and limitations. The recent demonstration that cancer immunotherapy extends patient survival has reinvigorated interest in elucidating the role ...
Experimental design Choice of mouse model. ... The role of mouse tumour models in the discovery and development of anticancer drugs. Br. J. Cancer 121, 101-108 (2019).
Experimental mouse tumour models have provided key mechanistic insights into host antitumour immune responses, and these have guided the development of novel treatment strategies. To accelerate ...
LIMITATIONS OF MOUSE TUMOUR MODELS. There is no doubt that experimental tumour models have contributed significantly in the understanding of molecular and other mechanisms of cancer development and treatment. Nevertheless, it has also been increasingly acknowledged that some fundamental shortcomings of preclinical models preclude the direct ...
In this paper, we have reviewed recent research progress in relation to mouse and rat models, focusing on spontaneous, induced, transgenic, and transplantable tumor models, to help guide the future study of malignant mechanisms and tumor prevention. Keywords: PDX; animal model; immune microenvironment; immunodeficiency; induced; spontaneity ...
Using a mutant mouse model of p53-3KR (K120R, K161R, and K162R), researchers found that Trp53 can still inhibit cancer initiation, mainly by regulating cell metabolism, despite losing the antitumor effect of inducing cell cycle arrest, apoptosis, and senescence (93).
The Experimental Mouse Shared Resource (EMSR) provides expertise to the University of Arizona Cancer Center and outside investigators since 1993. The EMSR offers a continuum of services including initial consultation and in vivo experimental design, Genetically Engineered Mouse (GEM) production, mouse experimentation and data analysis. The EMSR ...
Humanized mouse models of cancer are immunodeficient mice co-engrafted with human tumours and immune cells, and are used in immuno-oncology research with potential for clinical translation.
Experimental models: Cell lines: Human: 293T cell: Shanghai Cell Bank: Cat# SCSP-502: Mouse: Ctnnb1+Pten (CT) cell: This paper: N/A: Mouse: Trp53+Pten (PT) cell: ... Mouse liver cancer tissue and subcutaneous tumor tissue were ground and digested using a mouse tumor dissociation kit (Miltenyi Biotec, 130-096-730) and gentleMACS Dissociator ...
This review focuses only on the available mouse models for HCC research, because of the existence of a broad range of (i) chemically induced models, (ii) xenograft models and (iii) genetically modified models, in mice. The advantages and disadvantages of the three types of experimental mice models will be discussed in this review.
Lung cancer continues to have one of the highest morbidity and mortality rates of any cancer. Although radiochemotherapy, in combination with immunotherapy, has significantly improved overall survival, new treatment options are urgently needed. However, preclinical radiotherapy testing is often performed in animal models, which has several drawbacks, including species-specific differences and ...
In this protocol, mice are inoculated with two separate tumors derived from the same cell line. One tumor is removed and assessed before treatment; the other is used to assess the effect of treatment.
Breast cancer is the most commonly diagnosed cancer in women in the United States with 287,850 new cases and 43,250 deaths from this disease reported in 2022 ().Recent advances in the treatment of breast cancer have had a marked effect on overall outcomes although most of the benefit is seen in patients with early/localized breast cancer ().There remains a substantial need for new approaches ...
The Mouse Tumor Biology database (MTB) provides a publicly accessible electronic information resource for cancer researchers worldwide to both explore and contribute to this ever-expanding base of cancer biology data 13 - 15. Figure 1. Publications for mouse models of cancer. This graph was generated from data held in PubMed.
In a mouse tumor model, treatment with an HSP90 inhibitor suppressed tumor growth and reduced TAMs in the tumor microenvironment. ... We first established an experimental system to confirm that ...
Mouse Models of Breast Cancer Share Amplification and Deletion Events with Human Breast Cancer. J Mammary Gland Biol Neoplasia. 2017;22:71-84. Article PubMed PubMed Central Google Scholar