Practical Biology
A collection of experiments that demonstrate biological concepts and processes.
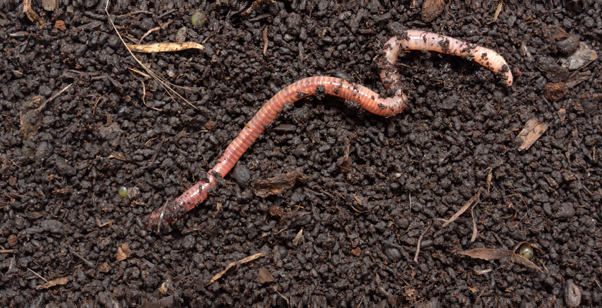

Observing earthworm locomotion
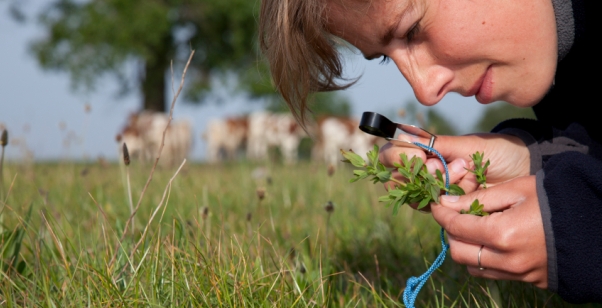
Practical Work for Learning
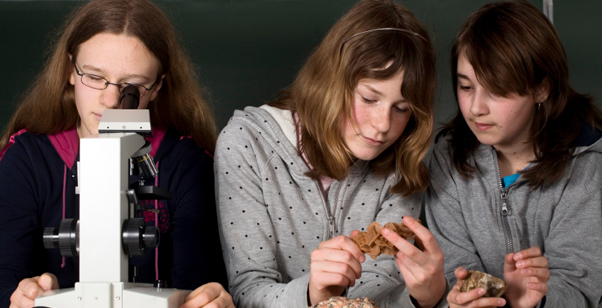
Published experiments
Investigating an enzyme-controlled reaction: catalase and hydrogen peroxide concentration, class practical or demonstration.
Hydrogen peroxide ( H 2 O 2 ) is a by-product of respiration and is made in all living cells. Hydrogen peroxide is harmful and must be removed as soon as it is produced in the cell. Cells make the enzyme catalase to remove hydrogen peroxide.
This investigation looks at the rate of oxygen production by the catalase in pureed potato as the concentration of hydrogen peroxide varies. The oxygen produced in 30 seconds is collected over water. Then the rate of reaction is calculated.
Lesson organisation
You could run this investigation as a demonstration at two different concentrations, or with groups of students each working with a different concentration of hydrogen peroxide. Individual students may then have time to gather repeat data. Groups of three could work to collect results for 5 different concentrations and rotate the roles of apparatus manipulator, result reader and scribe. Collating and comparing class results allows students to look for anomalous and inconsistent data.
Apparatus and Chemicals
For each group of students:.
Pneumatic trough/ plastic bowl/ access to suitable sink of water
Conical flask, 100 cm 3 , 2
Syringe (2 cm 3 ) to fit the second hole of the rubber bung, 1
Measuring cylinder, 100 cm 3 , 1
Measuring cylinder, 50 cm 3 , 1
Clamp stand, boss and clamp, 2
Stopclock/ stopwatch
For the class – set up by technician/ teacher:
Hydrogen peroxide, range of concentrations, 10 vol, 15 vol, 20 vol, 25 vol, and 30 vol, 2 cm 3 per group of each concentration ( Note 1 )
Pureed potato, fresh, in beaker with syringe to measure at least 20 cm 3 , 20 cm 3 per group per concentration of peroxide investigated ( Note 2 )
Rubber bung, 2-holed, to fit 100 cm 3 conical flasks – delivery tube in one hole (connected to 50 cm rubber tubing)
Health & Safety and Technical notes
Wear eye protection and cover clothing when handling hydrogen peroxide. Wash splashes of pureed potato or peroxide off the skin immediately. Be aware of pressure building up if reaction vessels become blocked. Take care inserting the bung in the conical flask – it needs to be a tight fit, so push and twist the bung in with care.
Read our standard health & safety guidance
1 Hydrogen peroxide: (See CLEAPSS Hazcard) Solutions less than 18 vol are LOW HAZARD. Solutions at concentrations of 18-28 vol are IRRITANT. Take care when removing the cap of the reagent bottle, as gas pressure may have built up inside. Dilute immediately before use and put in a clean brown bottle, because dilution also dilutes the decomposition inhibitor. Keep in brown bottles because hydrogen peroxide degrades faster in the light. Discard all unused solution. Do not return solution to stock bottles, because contaminants may cause decomposition and the stock bottle may explode after a time.
2 Pureed potato may irritate some people’s skin. Make fresh for each lesson, because catalase activity reduces noticeably over 2/3 hours. You might need to add water to make it less viscous and easier to use. Discs of potato react too slowly.
3 If the bubbles from the rubber tubing are too big, insert a glass pipette or glass tubing into the end of the rubber tube.
SAFETY: Wear eye protection and protect clothing from hydrogen peroxide. Rinse splashes of peroxide and pureed potato off the skin as quickly as possible.
Preparation
a Make just enough diluted hydrogen peroxide just before the lesson. Set out in brown bottles ( Note 1 ).
b Make pureed potato fresh for each lesson ( Note 2 ).
c Make up 2-holed bungs as described in apparatus list and in diagram.
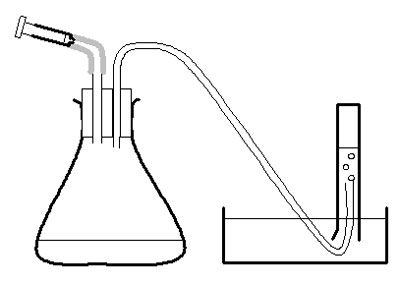
Investigation
d Use the large syringe to measure 20 cm 3 pureed potato into the conical flask.
e Put the bung securely in the flask – twist and push carefully.
f Half-fill the trough, bowl or sink with water.
g Fill the 50 cm 3 measuring cylinder with water. Invert it over the trough of water, with the open end under the surface of the water in the bowl, and with the end of the rubber tubing in the measuring cylinder. Clamp in place.
h Measure 2 cm 3 of hydrogen peroxide into the 2 cm 3 syringe. Put the syringe in place in the bung of the flask, but do not push the plunger straight away.
i Check the rubber tube is safely in the measuring cylinder. Push the plunger on the syringe and immediately start the stopclock.
j After 30 seconds, note the volume of oxygen in the measuring cylinder in a suitable table of results. ( Note 3 .)
k Empty and rinse the conical flask. Measure another 20 cm 3 pureed potato into it. Reassemble the apparatus, refill the measuring cylinder, and repeat from g to j with another concentration of hydrogen peroxide. Use a 100 cm 3 measuring cylinder for concentrations of hydrogen peroxide over 20 vol.
l Calculate the rate of oxygen production in cm 3 /s.
m Plot a graph of rate of oxygen production against concentration of hydrogen peroxide.
Teaching notes
Note the units for measuring the concentration of hydrogen peroxide – these are not SI units. 10 vol hydrogen peroxide will produce 10 cm 3 of oxygen from every cm 3 that decomposes.( Note 1 .)
In this procedure, 2 cm 3 of 10 vol hydrogen peroxide will release 20 cm 3 of oxygen if the reaction goes to completion. 2 cm 3 of liquid are added to the flask each time. So if the apparatus is free of leaks, 22 cm 3 of water should be displaced in the measuring cylinder with 10 vol hydrogen peroxide. Oxygen is soluble in water, but dissolves only slowly in water at normal room temperatures.
Use this information as a check on the practical set-up. Values below 22 cm 3 show that oxygen has escaped, or the hydrogen peroxide has not fully reacted, or the hydrogen peroxide concentration is not as expected. Ask students to explain how values over 22 cm 3 could happen.
An error of ± 0.05 cm 3 in measuring out 30 vol hydrogen peroxide could make an error of ± 1.5 cm 3 in oxygen production.
Liver also contains catalase, but handling offal is more controversial with students and introduces a greater hygiene risk. Also, the reaction is so vigorous that bubbles of mixture can carry pieces of liver into the delivery tube.
If collecting the gas over water is complicated, and you have access to a 100 cm 3 gas syringe, you could collect the gas in that instead. Be sure to clamp the gas syringe securely but carefully.
The reaction is exothermic. Students may notice the heat if they put their hands on the conical flask. How will this affect the results?
Health and safety checked, September 2008
http://www.saps.org.uk/secondary/teaching-resources/293-student-sheet-24-microscale-investigations-with-catalase Microscale investigations with catalase – which has been transcribed onto this site at Investigating catalase activity in different plant tissues.
(Website accessed October 2011)
Choose an Account to Log In

Notifications
Science project, catalase and hydrogen peroxide experiment.
How do living cells interact with the environment around them? All living things possess catalysts , or substances within them that speed up chemical reactions and processes. Enzymes are molecules that enable the chemical reactions that occur in all living things on earth. In this catalase and hydrogen peroxide experiment, we will discover how enzymes act as catalysts by causing chemical reactions to occur more quickly within living things. Using a potato and hydrogen peroxide, we can observe how enzymes like catalase work to perform decomposition , or the breaking down, of other substances. Catalase works to speed up the decomposition of hydrogen peroxide into oxygen and water. We will also test how this process is affected by changes in the temperature of the potato. Is the process faster or slower when compared to the control experiment conducted at room temperature?
What happens when a potato is combined with hydrogen peroxide?
- Hydrogen peroxide
- Small glass beaker or cup
- Divide the potato into three roughly equal sections.
- Keep one section raw and at room temperature.
- Place another section in the freezer for at least 30 minutes.
- Boil the last section for at least 5 minutes.
- Chop and mash a small sample (about a tablespoon) of the room temperature potato and place into beaker or cup.
- Pour enough hydrogen peroxide into the cup so that potato is submerged and observe.
- Repeat steps 5 & 6 with the boiled and frozen potato sections.
Observations & Results
Watch each of the potato/hydrogen peroxide mixtures and record what happens. The bubbling reaction you see is the metabolic process of decomposition , described earlier. This reaction is caused by catalase, an enzyme within the potato. You are observing catalase breaking hydrogen peroxide into oxygen and water. Which potato sample decomposed the most hydrogen peroxide? Which one reacted the least?
You should have noticed that the boiled potato produced little to no bubbles. This is because the heat degraded the catalase enzyme, making it incapable of processing the hydrogen peroxide. The frozen potato should have produced fewer bubbles than the room temperature sample because the cold temperature slowed the catalase enzyme’s ability to decompose the hydrogen peroxide. The room temperature potato produced the most bubbles because catalase works best at a room temperature.
Conclusions
Catalase acts as the catalyzing enzyme in the decomposition of hydrogen peroxide. Nearly all living things possess catalase, including us! This enzyme, like many others, aids in the decomposition of one substance into another. Catalase decomposes, or breaks down, hydrogen peroxide into water and oxygen.
Want to take a closer look? Go further in this experiment by looking at a very small sample of potato combined with hydrogen peroxide under a microscope!
Related learning resources
Add to collection, create new collection, new collection, new collection>, sign up to start collecting.
Bookmark this to easily find it later. Then send your curated collection to your children, or put together your own custom lesson plan.
Your browser is not supported
Sorry but it looks as if your browser is out of date. To get the best experience using our site we recommend that you upgrade or switch browsers.
Find a solution
- Skip to main content
- Skip to navigation
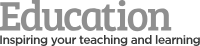
- Back to parent navigation item
- Primary teacher
- Secondary/FE teacher
- Early career or student teacher
- Higher education
- Curriculum support
- Literacy in science teaching
- Periodic table
- Interactive periodic table
- Climate change and sustainability
- Resources shop
- Collections
- Remote teaching support
- Starters for ten
- Screen experiments
- Assessment for learning
- Microscale chemistry
- Faces of chemistry
- Classic chemistry experiments
- Nuffield practical collection
- Anecdotes for chemistry teachers
- On this day in chemistry
- Global experiments
- PhET interactive simulations
- Chemistry vignettes
- Context and problem based learning
- Journal of the month
- Chemistry and art
- Art analysis
- Pigments and colours
- Ancient art: today's technology
- Psychology and art theory
- Art and archaeology
- Artists as chemists
- The physics of restoration and conservation
- Ancient Egyptian art
- Ancient Greek art
- Ancient Roman art
- Classic chemistry demonstrations
- In search of solutions
- In search of more solutions
- Creative problem-solving in chemistry
- Solar spark
- Chemistry for non-specialists
- Health and safety in higher education
- Analytical chemistry introductions
- Exhibition chemistry
- Introductory maths for higher education
- Commercial skills for chemists
- Kitchen chemistry
- Journals how to guides
- Chemistry in health
- Chemistry in sport
- Chemistry in your cupboard
- Chocolate chemistry
- Adnoddau addysgu cemeg Cymraeg
- The chemistry of fireworks
- Festive chemistry
- Education in Chemistry
- Teach Chemistry
- On-demand online
- Live online
- Selected PD articles
- PD for primary teachers
- PD for secondary teachers
- What we offer
- Chartered Science Teacher (CSciTeach)
- Teacher mentoring
- UK Chemistry Olympiad
- Who can enter?
- How does it work?
- Resources and past papers
- Top of the Bench
- Schools' Analyst
- Regional support
- Education coordinators
- RSC Yusuf Hamied Inspirational Science Programme
- RSC Education News
- Supporting teacher training
- Interest groups
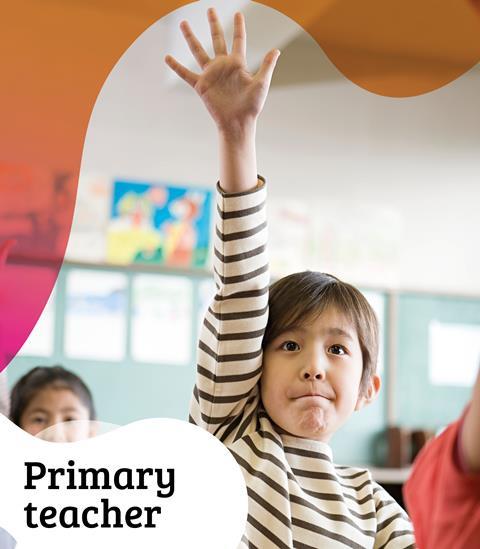
- More navigation items
Testing for catalase enzymes
In association with Nuffield Foundation
- No comments
Try this class experiment to detect the presence of enzymes as they catalyse the decomposition of hydrogen peroxide
Enzymes are biological catalysts which increase the speed of a chemical reaction. They are large protein molecules and are very specific to certain reactions. Hydrogen peroxide decomposes slowly in light to produce oxygen and water. The enzyme catalase can speed up (catalyse) this reaction.
In this practical, students investigate the presence of enzymes in liver, potato and celery by detecting the oxygen gas produced when hydrogen peroxide decomposes. The experiment should take no more than 20–30 minutes.
- Eye protection
- Conical flasks, 100 cm 3 , x3
- Measuring cylinder, 25 cm 3
- Bunsen burner
- Wooden splint
- A bucket or bin for disposal of waste materials
- Hydrogen peroxide solution, ‘5 volume’
Health, safety and technical notes
- Read our standard health and safety guidance.
- Wear eye protection throughout. Students must be instructed NOT to taste or eat any of the foods used in the experiment.
- Hydrogen peroxide solution, H 2 O 2 (aq) – see CLEAPSS Hazcard HC050 and CLEAPSS Recipe Book RB045. Hydrogen peroxide solution of ‘5 volume’ concentration is low hazard, but it will probably need to be prepared by dilution of a more concentrated solution which may be hazardous.
- Only small samples of liver, potato and celery are required. These should be prepared for the lesson ready to be used by students. A disposal bin or bucket for used samples should be provided to avoid these being put down the sink.
- Measure 25 cm 3 of hydrogen peroxide solution into each of three conical flasks.
- At the same time, add a small piece of liver to the first flask, a small piece of potato to the second flask, and a small piece of celery to the third flask.
- Hold a glowing splint in the neck of each flask.
- Note the time taken before each glowing splint is relit by the evolved oxygen.
- Dispose of all mixtures into the bucket or bin provided.
Teaching notes
Some vegetarian students may wish to opt out of handling liver samples, and this should be respected.
Before or after the experiment, the term enzyme will need to be introduced. The term may have been met previously in biological topics, but the notion that they act as catalysts and increase the rate of reactions may be new. Similarly their nature as large protein molecules whose catalytic activity can be very specific to certain chemical reactions may be unfamiliar. The name catalase for the enzyme present in all these foodstuffs can be introduced.
To show the similarity between enzymes and chemical catalysts, the teacher may wish to demonstrate (or ask the class to perform as part of the class experiment) the catalytic decomposition of hydrogen peroxide solution by manganese(IV) oxide (HARMFUL – see CLEAPSS Hazcard HC060).
If students have not performed the glowing splint test for oxygen for some time, they may need reminding of how to do so by a quick demonstration by the teacher.
More resources
Add context and inspire your learners with our short career videos showing how chemistry is making a difference .
Additional information
This is a resource from the Practical Chemistry project , developed by the Nuffield Foundation and the Royal Society of Chemistry.
Practical Chemistry activities accompany Practical Physics and Practical Biology .
© Nuffield Foundation and the Royal Society of Chemistry
- 11-14 years
- 14-16 years
- 16-18 years
- Practical experiments
- Biological chemistry
Specification
- Enzymes act as catalysts in biological systems.
- Factors which affect the rates of chemical reactions include: the concentrations of reactants in solution, the pressure of reacting gases, the surface area of solid reactants, the temperature and the presence of catalysts.
- Describe the characteristics of catalysts and their effect on rates of reaction.
- Recall that enzymes act as catalysts in biological systems.
- 7.6 Describe a catalyst as a substance that speeds up the rate of a reaction without altering the products of the reaction, being itself unchanged chemically and in mass at the end of the reaction
- 7.8 Recall that enzymes are biological catalysts and that enzymes are used in the production of alcoholic drinks
- C6.2.4 describe the characteristics of catalysts and their effect on rates of reaction
- C6.2.5 identify catalysts in reactions
- C6.2.14 describe the use of enzymes as catalysts in biological systems and some industrial processes
- C5.2f describe the characteristics of catalysts and their effect on rates of reaction
- C5.2i recall that enzymes act as catalysts in biological systems
- C6.2.13 describe the use of enzymes as catalysts in biological systems and some industrial processes
- C5.1f describe the characteristics of catalysts and their effect on rates of reaction
- C5.1i recall that enzymes act as catalysts in biological systems
- B2.24 The action of a catalyst, in terms of providing an alternative pathway with a lower activation energy.
- 2.3.5 demonstrate knowledge and understanding that a catalyst is a substance which increases the rate of a reaction without being used up and recall that transition metals and their compounds are often used as catalysts;
- 7. Investigate the effect of a number of variables on the rate of chemical reactions including the production of common gases and biochemical reactions.
Related articles
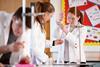
How to teach titration post-16
2024-07-08T05:32:00Z By Jo Haywood
Tips for teaching practical titration techniques and the underlying theory
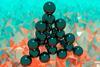
New method grows larger diamonds
2024-05-31T08:24:00Z By Nina Notman
Use this real-world context when teaching about giant covalent structures
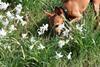
Why are some plants poisonous to you and your pets?
2024-05-22T08:16:00Z By Kit Chapman
Dig up the toxic secrets of nature’s blooms
No comments yet
Only registered users can comment on this article., more experiments.
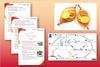
‘Gold’ coins on a microscale | 14–16 years
By Dorothy Warren and Sandrine Bouchelkia
Practical experiment where learners produce ‘gold’ coins by electroplating a copper coin with zinc, includes follow-up worksheet
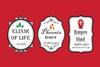
Practical potions microscale | 11–14 years
By Kirsty Patterson
Observe chemical changes in this microscale experiment with a spooky twist.
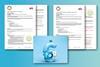
AP Sample 4 Lab 2 – Enzyme Catalysis
Introduction:
This lab will observe the conversion of hydrogen peroxide to water and oxygen gas by the enzyme catalysis. The amount of oxygen generated will be measured and used to calculate the rate of the enzyme-catalized reaction. Enzymes are proteins produced by living cells. Enzymes act as biochemical catalysts during a reaction, meaning they lower the activation energy needed for that reaction to occur. Through enzyme activity, cells gain the ability to carry out complex chemical activities at relatively low temperatures. The substance in an enzyme-catalyzed reaction that is to be acted upon is the substrate, which binds reversibly to the active site of the enzyme. The active site is the portion of the enzyme that interacts with the substrate. One result of this temporary union between the substrate and the active site is a reduction in the activation energy required to start the reaction of the substrate molecule so that products are formed. In a mathematical equation of the substrate (S) binding with the activation site (E) and forming products (P) is:
E + S —> ES –> E + P
Several ways enzyme action may be affected include:
1) Salt Concentration — For example, if salt concentration is close to zero, the charged amino acid side chains of the enzyme molecules will attract each other. The enzyme will then denature and form an inactive precipitate. If salt concentration is extremely high, the normal interaction of charged groups will be blocked, new interactions will occur, and again the enzyme will precipitate. An intermediate salt concentration such as that of human blood (0.9%) is the optimum for many enzymes.
2) pH of the environment — . The pH of a solution is a logarithmic scale that measures the acidity or H+ concentration in a solution. The scale begins at 0, being the highest in acidity, and ends at 14, containing the least amount of acidity. As the pH is lowered an enzyme will tend to gain H+ ions, disrupting the enzyme’s shape. In turn, if the pH is raised, the enzyme will lose H+ ions and eventually lose its active shape.
3) Temperature — Usually, chemical reactions speed up as the temperature is raised. When the temperature is increased, more of the reacting molecules have enough kinetic energy to undergo the reaction. However, if the temperature goes past a temperature optimum, the conformation of the enzyme molecules is disrupted.
4) Activations and Inhibitors — Many molecules other than the substrate may interact with an enzyme. If such a molecule speeds up the reaction it is an activator, but if it slows the reaction down it is an inhibitor.
The enzyme used in this lab is catalase, which has four polypeptide chains that are composed of more than 500 amino acids each. One function of this enzyme is to prevent the accumulation of toxic levels of hydrogen peroxide formed as a by-product of metabolic processes. Catalase is also involved in some of the many oxidation reactions that occur in the cells of all living things. The primary reaction catalyzed by catalase is the decomposition of hydrogen peroxide to form water and oxygen.
2H2O2 ——-> 2 H2O + O2 (gas)
Without the help of catalase, this reaction occurs spontaneously, but very slowly. Catalase helps to speed up the reaction considerably. In this lab, a rate for this reaction will be determined.
Hypothesis:
The enzyme catalase, under optimum salt conditions, temperature, and pH level will speed up the reaction as it denatures the hydrogen peroxide at a higher rate than normal.
Exercise 2A
For the first part of the lab, 10 mL of 1.5% H2O2, a 50-mL glass beaker, and 1 mL of fresh catalase are needed. At the second stage a test tube, a hot water bath, 5 mL of catalase, 10 mL of 1.5% H2O2 are needed. Finally, in the third part, a potato, and10 mL of 1.5% H2O2 are needed.
Exercise 2B
For this experiment, 10 mL of 1.5% H2O2, 1 mL of water, 10 mL of sulfuric acid, two 25 mL beakers, 5-10 mL syringe, potassium permanganate, lab aprons and trays are needed.
Exercise 2C
In this section of the experiment, 20 mL of 1.5% H2O2, two glass beakers, 1 mL of H2O, 10 mL of sulfuric acid, a 5 mL syringe, 5-10 mL of potassium permanganate, and lab aprons and trays are used.
Exercise 2D
In the final part of the lab, 6 plastic cups labeled 10, 30, 60, 120, 180, 360, 6 plastic cups labeled acid, 60 mL of 1.5% H2O2, a 50-mL beaker, 6 mL of catalase extract, two 5-mL syringes, potassium permanganate, a timer (clock), lab aprons and trays are needed.
Transfer 10 mL of 1.5% H2O2 into a 50-mL glass beaker and add 1 mL of freshly made catalase solution. The fresh catalase should be kept on ice until ready to be used. Observe the reaction. Then transfer 5 mL of purified catalase extract to a test tube and place it in a hot water bath for five minutes. Transfer 10 mL of 1.5% H2O2 into a 50-mL beaker and add 1 mL of the boiled catalase solution, after it has cooled. Observe the changes in the reaction. To demonstrate the presence of catalase in living tissue, cut 1 cubic cm of potato, macerate it, and transfer it into a 50-mL glass beaker containing 10 mL of 1.5% H2O2. Observe the results.
Put 10 ml of 1.5% H2O2 into a clean glass beaker. Add 1 mL of H2O. Add 10 mL of sulfuric acid (1.0 M). USE EXTREME CARE IN HANDLING ACIDS. Mix the solution well. Remove a 5 mL sample. Place this 5 mL sample in another beaker, and assay for the amount of H2O2 as follows: Place the beaker containing the sample over white paper. Use a burette or 5 mL pipette to add potassium permanganate a drop at a time to the solution until a persistent pink or brown color is obtained. Remember to gently swirl the solution after adding each drop.
To determine the rate of spontaneous conversion of H2O2 to H2O and O2in an uncatalyzed reaction, put about 20 mL of 1.5% H2O2 in a beaker. Store it uncovered at room temperature for approximately 24 hours. Put 10 mL of 1.5% H2O2 into a clean glass beaker (using the uncatalyzed H2O2 that set out). Add 1 mL of H2O2 and then add 10 mL of sulfuric acid (1.0 M). Be careful when using acid. Mix this solution well. Remove a 5 mL sample and place it into another beaker. Assay for the amount of H2O2 as follows: Use a 5 mL syringe to add one drop of potassium permanganate at a time to the solution until it becomes a persistent pink or brown color. Gently swirl the solution after adding each drop. Record all results.
If a day or more has passed since Exercise B was performed, a baseline must be reestablish. Repeat the assay from Exercise B and record the results. Compare with other groups to check that results are similar. To determine the course of an enzymatic reaction, how much substrate is disappearing over time must be measured. The first thing to be done is to set up the six cups labeled with times, and the other six, one directly in front of each cup with a time on it. Then put 10 mL of 1.5% H2O2 into the cup marked 10 sec. Add 1 mL of catalase extract to this cup. Swirl gently for 10 seconds using a timer or clock for help. At 10 seconds, add 10 mL of sulfuric acid. Remove 5 mL and place in the cup directly in front of the cup marked 10 sec. Assay the 5 mL sample by adding one drop of potassium permanganate at a time until the solution turns a pink or brown. Repeat the previous steps, with clean cups using the times 30, 60, 120, 180, and 360. Record all results and observations.
Enzyme Activity
Activity | |
The reaction caused oxygen gas as a product, which made the wooden splint glow bright red. | |
| Boiling the catalase caused it to denature and it resulted in no bubbling in the solution. |
The catalase being present in a living thing (potato), caused an extreme reaction with tons of products (02) produced. |
Establishing a Baseline
| 10 mL |
| 6.7 mL |
Baseline ( final volume – initial volume)
| 3.3 mL Potassium permanganate |
Rate of Hydrogen Peroxide Spontaneous Decomposition
Initial KMnO4
| 5 mL |
| .3 mL |
Amount of KMnO4 used after 24 hours
| 4.7 mL |
Amount of H2O2 spontaneously decomposed ( ml baseline – ml after 24 hours) | 1.4 mL |
( ml baseline – ml after 24 hours/ baseline) | 57.6% |
Reestablishing a Baseline
| 5 mL |
| .8 mL |
Baseline ( final volume – initial volume)
| 4.2 mL Potassium permanganate |
Rate of Hydrogen Peroxide Decomposition by Catalase
| ||||||
Baseline KMnO4 | 4.2 | 4.2 | 4.2 | 4.2 | 4.2 | 4.2 |
Initial volume KMnO4
| 10 | 10 | 10 | 10 | 10 | 10 |
Final volume KMnO4
| 7.1 | 7.9 | 8.1 | 8.5 | 9.2 | 9.4 |
Amount KMnO4 used (baseline – final) | 2.9 | 2.1 | 1.9 | 1.5 | .8 | .6 |
(KMnO4 – initial)
| 1.3 | 2.1 | 2.3 | 2.7 | 3.4 | 3.6 |
1. a) What is the enzyme in this reaction? Catalase is the enzyme in the reaction.
b) What is the substrate in this reaction? The substrate is hydrogen peroxide.
c) What is the product in this reaction? The products are oxygen (gas) and water.
d) How could you show that the gas evolved is oxygen? Using the example of holding a burning wooden splint over the reaction, the splint glows bright red, therefore showing that oxygen is being let out of the solution.
2. How does the reaction compare to the one using the unboiled catalase? Explain the reason for this difference. When the boiled catalase was used, there was no bubbling in the solution, which proved that there was no reaction occurring because the extreme heat had denatured the catalase.
3. What do you observe? What do you think would happen if the potato or liver was boiled before being added to the hydrogen peroxide? The catalase shows a lot of reaction with the potato, causing many bubbles to form in the solution. Also, if the potato were boiled there wouldn’t be any bubbles, because the heat would denature the potato.
Analysis of Results
1. Determine the initial rate of the reaction and the rates between each of the time points. Record the rates in the table below.
.13 | .04 | .007 | .007 | .0012 | .0011 |
2. When is the rate the highest? Explain why. The rate is the highest at the beginning of the reaction, because the hydrogen peroxide had been exposed to the air for the least amount of time.
3. When is the rate the lowest? For what reasons is the rate low? At the longer times the rate was the lowest because the peroxide had been exposed to the air longer.
4. Explain the inhibiting effect of sulfuric acid on the function of catalase. Relate this to enzyme structure and chemistry. The sulfuric acid lowered the pH level of the solution, which caused the catalase to denature by gaining hydrogen ions and it stopped the reaction immediately.
5. Predict the effect lowering the temperature would have on the rate of enzyme activity. Explain you prediction. Enzymes work best at optimum temperature, therefore increasing, or in this case, decreasing the temperature would extremely change the rate of the reaction. Lowering the temperature would cause the reaction to slow down.
6. Design a controlled experiment to test the effect of varying pH, temperature, or enzyme concentration. Since the results of room temperature and heated have already been recorded, using catalase that was completely frozen would test the other end of the spectrum as far as temperature goes.
Error Analysis:
Any errors occurring in this experiment could have been caused by misreading of a syringe, miscalculating the data on the tables, or when the 1.5% H2O2 was mixed that was used in almost all parts of the experiment. Also, in Exercise 2D, if the getting the timing just right with all parts of the lab were a source of error in the experiment.
Discussion and Conclusion:
The main purpose of this lab was to show how enzymes can be affected in reactions with other substances by factors such as pH, temperature, and exposure to the surrounding environment. This lab proved that an extreme increase in temperature (boiling) can cause absolutely no reaction by using the boiled enzyme catalase. Also, by using a potato, it shows that catalase is speeding up the decomposition of hydrogen peroxide in living things, helping all living things survive another day.
SITEMAP * HOME PAGE * SEARCH * UK KS3 level Science Quizzes for students aged ~13-14
UK GCSE level Biology * Chemistry * Physics ~14-16 * Advanced pre-university Chemistry ~16-18
UK GCSE level age ~14-16, ~US grades 9-10 Biology revision notes
Doc Brown's Biology exam study revision notes There are various sections to work through, after 1 they can be read and studied in any order. Download of all my science revision notes and quizzes (Read notes first) Here, the product oxygen gas, provides the means of following the rate of the reaction. Enzyme reaction equation: hydrogen peroxide === catalase ===> water + (gas) 2H O (aq) ====> 2H O(l) + (g)
The basic procedures for
(vary either the hydrogen peroxide or the enzyme catalase). Enzyme reaction equation: hydrogen peroxide === catalase ===> water + (gas) 2H O (aq) ====> 2H O(l) + (g) Mashed up potato made into a fine diluted with acts as a source of the enzyme catalase. It needs to be well shaken before use so that each portion measured out has the same amount of catalase in it. is a pulverized solid mixed in a liquid.You need a series of hydrogen peroxide solutions of known different concentrations and a fixed concentration of the potato mix to investigate the effect of changing the hydrogen peroxide concentration. You can also keep the hydrogen peroxide concentration constant and do the investigation with a set of different concentrations of the potato-catalase mixture. The water bath is set to a constant temperature e.g. 25 C. The apparatus is setup as illustrated above. for human catalase are pH 7 (so no need for buffer) and 37 CIf no thermostated water bath is available you can get reasonable results if the laboratory temperature stays reasonably constant - but record and monitor the room temperature. The 'stock' solutions of potato-catalase or hydrogen peroxide should be initially in separate boiling tubes and placed in the water bath so that everything starts at the right temperature. Or, if no water bath available, they can be just put together in test tube racks on the laboratory bench, but you should monitor and record the room temperature. Depending on what accuracy you require, you can measure out a fixed amount of the potato slurry and a varied amount of the hydrogen peroxide solution into the boiling tube using a pipette, or 10 cm measuring cylinder or plastic syringe. If in doubt build a fixed volume of a pH 7 buffer into your method.
You should make up the reaction mixture of hydrogen peroxide and potato slurry as quickly as possible and shake well. Your reaction mixture to vary the hydrogen peroxide concentration may be as follows of buffer (if used)x cm of potato slurry - kept constant y cm of hydrogen peroxide solution - variable z cm of water - variable You can vary y and z to give different concentrations if different stock solutions of By varying volumes y and z you can produce a range of hydrogen peroxide concentrations if a variety of stock solutions are not available.. The boiling tube and mixture is quickly connected to the delivery tube rubber bung and placed in the water bath and the stop watch started. Make sure the boiling tube is fully immersed in water so it and the contents are at the right temperature. Start the stopwatch. You can now measure how much oxygen is formed in a set time e.g. 1 minute, and repeat the experiment several times with the same volumes of reactants at the same temperature. This will allow a more accurate mean value of the rate of reaction to be used in the final analysis.
However you get the results, the : From the initial gradient of the graph, the rate of enzyme reaction is expressed as: formed � time taken (cm /s)You then draw a graph of the mean values of the rate of reaction (in cm /s) at each temperature versus concentration. You should find that the rate increases with increase in either hydrogen peroxide or enzyme concentration, if you have been very accurate you may get a nice linear graph like the one on the right or else! You then repeat the whole exercise with different concentrations of the enzyme using the kind of x + y + z 'recipe' described above using a fixed concentration of hydrogen peroxide. The investigation is conducted in the same way as already described above.
Graph line A (steeper gradient) compared to graph line B may represent (i) an increase in concentration of either substrate or enzyme, or (ii) an increase in temperature or (iii) a solution pH nearer the optimum value for that particular enzyme. Investigating the effect of changing temperature for an enzyme reaction Enzyme reaction equation: hydrogen peroxide === catalase ===> water + (gas) 2H O (aq) ====> 2H O(l) + (g) Mashed up potato made into a fine slurry diluted with water acts as a source of the enzyme catalase. It needs to be well shaken before use so that each portion measured out has the same amount of catalase in it. You need a hydrogen peroxide solution of known and constant concentration. You should also use the same volume of the well shaken potato slurry. The water bath is set to the start temperature e.g. 20 C. The apparatus setup is illustrated above. C perhaps by cooling the water with ice, not sure how well it would work?The two 'stock' solutions should be initially in separate boiling tubes and placed in the water bath so that everything starts at the right temperature - solutions and boiling tube. Depending on what accuracy you require, you can measure out a fixed amount of the potato slurry and a fixed amount of the same hydrogen peroxide solution into the boiling tube using a pipette or a 10 cm measuring cylinder or plastic syringe. You should keep the total volume of reaction mixture constant. (If in doubt use a buffer to match the optimum pH of the enzyme catalase).
You make up the reaction mixtures as quickly as possible in a boiling tube and shake well. The boiling tube and mixture is quickly connected to the delivery tube rubber bung and the stop watch started. Make sure the boiling tube is fully immersed in water so it and the contents are at the right temperature.
Repeats will allow a more accurate average value of the rate of reaction to be used in the final analysis. (or set of volume readings for one run, plot graph of volume versus time and measure the initial gradient, but more work for repeats - see the graph on the right) From the initial gradient of the graph, the rate of enzyme reaction is expressed as: formed/time taken (cm /s)C, 40 C, 50 C etc. adjusting the thermostat temperature control. You should find from 20 C to 40 C an increase in the rate of oxygen production, but an increasingly slower rate of reaction from 50 C to 70 C (see graph on bottom right). You then draw a graph of the mean values of the rate of reaction (in cm /s) at each temperature versus temperature.
Investigating the effect of changing pH for an enzyme reaction Enzyme reaction equation: hydrogen peroxide === catalase ===> water + (gas) 2H O (aq) ====> 2H O(l) + (g) Mashed up potato made into a fine slurry diluted with water acts as a source of the enzyme catalase. It needs to be well shaken before use so that each portion measured out has the same amount of catalase in it. You need a hydrogen peroxide solution of known and constant concentration AND stock solution of the potato slurry to provide the enzyme catalase. You need a range of at least five stock solutions of buffers giving a variety of pH values e.g. ideally from pH 2 to pH 11. A buffer solution keeps the pH constant in a reaction medium - it can neutralise small amounts of acid or alkali formed. The water bath is set to a e.g. 25 C-35 C. C).
If no thermostated water bath is available you can get reasonable results if the laboratory temperature stays reasonably constant - measure and monitor. The 'stock' solutions of catalase, hydrogen peroxide and the buffer solutions should be initially in separate boiling tubes and placed in the water bath so that everything starts at the right temperature. Or, if no water bath available, they can be just together in test tube racks on the laboratory bench, but you should monitor and record the room temperature. Depending on what accuracy you require, you can measure out a fixed amounts of the potato slurry, hydrogen peroxide solution and the buffer solutions into the boiling tube using a pipette or more accurately with a 10 cm measuring cylinder. The three solutions are mixed in a boiling tube and well mixed, the total volume should be constant and you use the same concentrations of the hydrogen peroxide and potato-catalase. The pH of the buffer should be the only variable. The boiling tube and mixture is quickly connected to the delivery tube rubber bung and the stop watch started. Make sure the boiling tube is fully immersed in water so it and the contents are at the right temperature.
This will allow a more accurate mean value of the rate of reaction to be used in the final analysis. (or set of volume readings for one run, plot graph of volume versus time and measure the initial gradient, but more work doing repeats - see the graph on the right) From the initial gradient of the graph, the rate of enzyme reaction is expressed as: formed/time taken (cm /s)
You then draw a graph of the mean values of the rate of reaction (in cm /s) versus the pH, and it 'may' look like the graph on the right. See the end of method 1. (a) for a Know about the experimental methods for investigating the enzyme catalysed decomposition of hydrogen peroxide by catalase and how to investigate the effects of changing temperature, changing pH and changing the concentration. Know what apparatus is needed and the main points of the experimental procedure for the enzyme decomposition of hydrogen peroxide, including the apparatus and chemicals required.
) ] below, maybe quicker than the indexes
Links to all indexes for KS3 science students aged ~11-14, ~US grades 6, 7 and 8* * UK GCSE level students aged ~14-16, ~US grades 9-10 for pre-university age ~16-18 ~US grades 11-12, K12 Honors Website content � Dr Phil Brown 2000+. All copyrights reserved on Doc Brown's biology revision notes, images, quizzes, worksheets etc. Copying of website material is NOT permitted. Exam revision summaries and references to science course specifications are unofficial. |
specific biology words or courses e.g. topic, module, exam board, structure, concept, animal or plant feature, 'phrase', homework question! anything of biological interest! This is a Google generated search of my website. |
TOP OF PAGE
Laboratory Manual For SCI103 Biology I at Roxbury Community College
Enzymes are macromolecular biological catalysts. The molecules upon which enzymes may act are called substrates and the enzyme converts the substrates into different molecules known as products. Almost all metabolic processes in the cell need enzyme catalysis in order to occur at rates fast enough to sustain life. Metabolic pathways depend upon enzymes to catalyze individual steps. Enzymes are known to catalyze more than 5,000 biochemical reaction types. Most enzymes are proteins, although a few are catalytic RNA molecules. The latter are called ribozymes. Enzymes’ specificity comes from their unique three-dimensional structures.
Like all catalysts, enzymes increase the reaction rate by lowering its activation energy. Some enzymes can make their conversion of substrate to product occur many millions of times faster. An extreme example is orotidine 5’-phosphate decarboxylase, which allows a reaction that would otherwise take millions of years to occur in milliseconds. Chemically, enzymes are like any catalyst and are not consumed in chemical reactions, nor do they alter the equilibrium of a reaction. Enzymes differ from most other catalysts by being much more specific. Enzyme activity can be affected by other molecules: inhibitors are molecules that decrease enzyme activity, and activators are molecules that increase activity. Many therapeutic drugs and poisons are enzyme inhibitors. An enzyme’s activity decreases markedly outside its optimal temperature and pH.
Some enzymes are used commercially, for example, in the synthesis of antibiotics. Some household products use enzymes to speed up chemical reactions: enzymes in biological washing powders break down protein, starch or fat stains on clothes, and enzymes in meat tenderizer break down proteins into smaller molecules, making the meat easier to chew.
In this laboratory, we will study the effect of temperature, concentration and pH and on the activity of the enzyme catalase. Catalase speeds up the following reaction:
2 H 2 O 2 -> 2 H 2 O + O 2
Hydrogen peroxide is toxic. Cells therefore use catalase to protect themselves. In these experiments, we will use catalase enzyme from potato.
The first experiment will establish that our catalase works (positive control) and that our reagents are not contaminated (negative control).
Hydrogen peroxide will not spontaneously degrade at room temperature in the absence of enzyme. When catalase is added to hydrogen peroxide, the reaction will take place and the oxygen produced will lead to the formation of bubbles in the solution. The height of the bubbles above the solution will be our measure of enzyme activity (Figure 8.2 ).
8.1 Positive and negative controls (Experiment 1)
8.1.1 experimental procedures.
- Obtain and label three 15 ml conical plastic reaction tubes.
- Add 1 ml of potato juice (catalase) to tube 1 (use a plastic transfer pipette).
- Add 4 ml of hydrogen peroxide to tube 1. Swirl well to mix and wait at least 20 seconds for bubbling to develop.
- Use a ruler (Figure 8.1 ) and measure the height of the bubble column above the liquid (in millimeters; use the centimeter scale of the ruler) and record the result in Table 8.1 .
- Add 1 ml of water.
- Add 4 ml of hydrogen peroxide. Swirl well to mix and wait at least 20 seconds.
- Measure the height of the bubble column (in millimeters) and record the result in Table 8.1 .
- Add 1 ml of potato juice (catalase).
- Add 4 ml of sucrose solution. Swirl well to mix; wait 20 seconds.
- Measure the height of the bubble column and record the result in Table 8.1 .
Tube # | Height of bubbles (mm) |
---|---|
1 | |
2 | |
3 |

Figure 8.1: A ruler with metric (cm) and imperial (inch) scales.

Figure 8.2: Results from experiment 1. Compare with your results!
8.2 Effect of temperature on enzyme activity (Experiment 2)
8.2.1 experimental procedures.
Before you begin with the actual experiment, write down in your own words the hypothesis for this experiment:
- Obtain and label three tubes.
- Add 1 ml of potato juice (catalase) to each tube.
- Place tube 1 in the refrigerator, tube 2 in a 37 °C (Celsius) heat block, and tube 3 in a 97 °C heat block for 15 minutes.
- Remove the tubes with the potato juice (catalase) from the refrigerator and heat blocks and immediately add 4 ml hydrogen peroxide to each tube.
- Swirl well to mix and wait 20 seconds.
- Measure the height of the bubble column (in millimeters) in each tube and record your observations in Table 8.2 .
Do the data support or contradict your hypothesis?
Tube # | Height of bubbles (mm) |
---|---|
1 | |
2 | |
3 |

Figure 8.3: Results from experiment 2. Compare with your results!
8.3 Effect of concentration on enzyme activity (Experiment 3)
8.3.1 experimental procedures.
- Measure the height of the bubble column (in millimeters) and record your observations in Table 8.3 .
- Add 3 ml of potato juice (catalase).
Tube # | Height of bubbles (mm) |
---|---|
1 | |
2 | |
3 |

Figure 8.4: Results from experiment 3. Compare with your results!
8.4 Effect of pH on enzyme activity (Experiment 4)
8.4.1 experimental procedures.
- Obtain 6 tubes and label each tube with a number from 1 to 6.
- Place the tubes from left (tube #1) to right (tube #6) in the first row of a test tube rack.
- Add to 1 ml of potato juice (catalase) to each tube.
- Add 2 ml of water to tube 1.
- Add 2 ml of pH buffer 3 to tube 2.
- Add 2 ml of pH buffer 5 to tube 3.
- Add 2 ml of pH buffer 7 to tube 4.
- Add 2 ml of pH buffer 9 to tube 5.
- Add 2 ml of pH buffer 12 to tube 6.
- Add 4 ml of hydrogen peroxide to each of the six tubes.
- Swirl each tube well to mix and wait at least 20 seconds.
- Measure the height of the bubble column (in millimeters) in each tube and record your observations in Table 8.4 .
Tube # | Height of bubbles (mm) |
---|---|
1 | |
2 | |
3 | |
4 | |
5 | |
6 |

Figure 8.5: Results from experiment 4. Compare with your results!
Figure 8.6: Catalase activity is dependent on pH. The data shown in this figure were obtained by three groups of students during a previous laboratory session. The triangles represent the data from the experimental results shown in Figure 8.5 .
8.5 Cleaning up
- Empty the contents of the plastic tubes into the labeled waste container (brown bottle) in the chemical fume hood.
- Discard the empty tubes and other waste in the regular waste basket.
- Rinse the glass rod and glassware with water and detergent.
- Return the glass ware to the trays on your bench where you originally found them.
8.6 Review Questions
- What is a catalyst?
- What are enzymes?
- What is the name of the enzyme that we studied in this laboratrory session?
- What is an enzyme substrate?
- What is the substrate of the enzyme that we used in this laborator seesion?
- What are the products of the reaction that was catalized by the enzyme that we studied in this laboratory seesion?
- What is the active site of an enzyme?
- What is the purpose of the negative and positive controls?
- State the hypothesis that was tested in experiment 2?
- State the hypothesis that was tested in experiment 3?
- State the hypothesis that was tested in experiment 4?
- The enzyme from potato appeared to work better at 4 °C than at 37 °C. Would you expect the same if we had used the equivalent human enzyme? Justify your answer.
- Why did heating the enzyme at high temperature (> 65 °C) result in loss of activity?
Search Filters:
Main Navigation
Search Cornell
Cornell Institute for Biology Teachers
- Labs & Activities
- Workshops & Events
- Equipment Lending Library
- Connect with Cornell
- Labs & Activities
Browse Labs & Activities:
- Abuse-a-Cyst- University of Utah
- Acid Rain Lab- Katherine Betrus Derrico
- Battle-jar Galactica- Matt Downing
- Becoming a Plant
- Biological Shapes
- Biomagnification Lab- Todd Shuskey
- Blood Vessel Physiology
- Bottle Ecosystem- Tim Downs
- Bouquet of Flowers
- Bovine Uterus Dissection
- Building Blocks of Life
- Case of the Missing Diamond Maker
- Comparative Skulls
- Comparing Aquatic Communities
- DNA Molecule Activity
- DNA Profiling
- Easy Stats Lab
- Edible Earth Parfaits- Groundwater Foundation
- Evolving Trees
- Fetal Development
- Floating Leaf Disk- Brad Williamson
- Food Chain Game- Delta Education
- Goldenrod Galls
- HIV Transmission
- How Many CATs
- I’ll Have a Bite! – Greg Panzanaro
- Insect GENEration
- Lichens on Tree Trunks- Scott LaGreca
- Mark-Recapture- Nancy Wright
- Medical Importance of Biodiversity- Mary Keymel
- Metric Measurement
- Microscopy and Cell Biology
- Mollusk Dichotomous Key
- Mystery of the (Hominin) Skulls
- Oh Deer- Project WILD
- Oil Spills Lab- Kristen Pizarro
- Predator-Prey Population Oscillation- Bridget Henshaw
- Primary Productivity Lab- modified by Sean McGlynn
- Protein Gel Electrophoresis
- Protein Synthesis and Words- Comet
- Pseudomonas Labs
- Radish Seed Lab- Chris Courtsunis
- Rotifers Lab- Beth Chagrasulis
- Shake and Break: An Earthquake Simulation
- Stomata Safari- Carolyn Wilczynski
- Tardigrade Lab
- Tell-Tale Heart
- Vegevaders- PPI
- Vocabulary and Story Writing- Karen Cook
- Web of Gold Activity- Robert Raguso
- World of Crickets
High School
Molecular biology.
The structure and function of enzymes is a central theme in cellular and molecular biology. In this laboratory exercise, a crude cell extract is prepared from potatoes.
Activity of the enzyme, catalase [which catalyzes the reaction 2H 2 O 2 (l) → 2H 2 O(l) + O 2 (g)], is then studied using a simple assay for O 2 . To conduct the assay, a filter is soaked in crude potato extract, then transferred to the bottom of a beaker containing hydrogen peroxide. Catalase causes O 2 to collect in the filter, which in turn causes the filter to rise. Students are able to explore the effect of enzyme and/or substrate concentration and pH on the amount of product formed by measuring the time taken for each filter to collect enough oxygen to rise. Students average their results, calculate the inverse of the “time to rise,” and pool the data in order to plot the characteristic curve showing the dependence of enzyme activity on substrate and enzyme concentration.
Catalase Teacher Edition (CIBT)
Catalase Student Edition (CIBT)
-->Cornell University --> ©2014 ↑
Enhanced Adsorption of Uranium (VI) from Aqueous Solutions by Hydrogen Peroxide-Modified Magnetic Biochar: Performance Evaluation and Mechanistic Study
- Published: 27 September 2024
- Volume 235 , article number 708 , ( 2024 )
Cite this article
- Zhenzhen Xu 1 ,
- Hangxiang Li 2 ,
- Yangyang Zhang ORCID: orcid.org/0000-0001-7222-3957 1 &
The improper disposal of uranium mine tailings and smelting wastewater poses significant environmental hazards, including contamination of water bodies and soil, and potential health risks to humans through the accumulation of uranium in bones and organs. This study examines the removal of hexavalent uranium (U(VI)) from water using biochar derived from agricultural waste enhanced by hydrogen peroxide (H 2 O 2 ) and magnetization modifications involving the incorporation of Fe 3 O 4 nanoparticles onto the biochar surface. The adsorption capacities were evaluated through batch experiments, and the mechanisms were analyzed using SEM, FT-IR, XPS, and BET surface analysis. These modifications were found to significantly increase the biochar's specific surface area from 17.606 m 2 /g to 195.62 m 2 /g and pore volume from 0.037 cm 3 /g to 0.132 cm 3 /g, and surface enrichment with oxygen-containing functional groups, particularly carboxyl groups. The inherent adsorption efficiency of biochar for U(VI) is significantly enhanced by these modifications, with the adsorption capacities of H 2 O 2 -modified (HBC) and magnetized HBC (MBC) reaching 69.50 mg/g and 77.58 mg/g, respectively. The adsorption mechanisms of BC, HBC, and MBC for U(VI) involve complexation reactions where hydroxyl and carboxyl groups, among other oxygen-containing functional groups on the biochar, interact with uranyl ions. HBC and MBC exhibit enhanced complexation due to higher carboxyl content, leading to improved U(VI) adsorption. Additionally, the presence of iron-containing functional groups on the surface of MBC contributes to electrostatic interactions and facilitates complexation reactions between Fe-O and uranyl ions, enhancing the overall adsorption process. These findings highlight the potential of modified biochar for the removal of U(VI) from water, particularly for the treatment of mine tailings and smelting wastewater, with significant implications for industrial applications in environmental remediation.
This is a preview of subscription content, log in via an institution to check access.
Access this article
Subscribe and save.
- Get 10 units per month
- Download Article/Chapter or eBook
- 1 Unit = 1 Article or 1 Chapter
- Cancel anytime
Price includes VAT (Russian Federation)
Instant access to the full article PDF.
Rent this article via DeepDyve
Institutional subscriptions
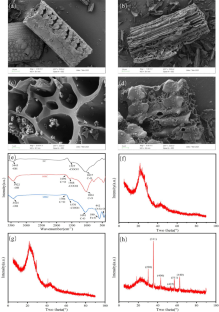
Explore related subjects
- Environmental Chemistry
Data Availability
The data that support the findings of this study are available from the corresponding author, upon request.
Ahmed, W., Mehmood, S., Qaswar, M., Ali, S., Khan, Z. H., Ying, H., Chen, D.-Y., & Núñez-Delgado, A. (2021). Oxidized biochar obtained from rice straw as adsorbent to remove uranium (VI) from aqueous solutions. Journal of Environmental Chemical Engineering, 9 , 105104.
Article CAS Google Scholar
Alessi, D. S., Uster, B., Veeramani, H., Suvorova, E. I., Lezama-Pacheco, J. S., Stubbs, J. E., Bargar, J. R., & Bernier-Latmani, R. (2012). Quantitative separation of monomeric U (IV) from UO 2 in products of U (VI) reduction. Environmental Science & Technology, 46 , 6150–6157.
Bindal, S. K., Nikolov, A. D., Wasan, D. T., Lambert, D. P., & Koopman, D. C. (2001). Foaming in simulated radioactive waste. Environmental Science & Technology, 35 , 3941–3947.
Bolan, N., Hoang, S. A., Beiyuan, J., Gupta, S., Hou, D., Karakoti, A., Joseph, S., Jung, S., Kim, K.-H., & Kirkham, M. (2022). Multifunctional applications of biochar beyond carbon storage. International Materials Reviews, 67 , 150–200.
Chen, X., Xia, H., Lv, J., Liu, Y., Li, Y., Xu, L., Xie, C., & Wang, Y. (2023). Magnetic hydrothermal biochar for efficient enrichment of uranium (VI) by embedding Fe 3 O 4 nanoparticles on bamboo materials from “one-can” strategy. Colloids and Surfaces A: Physicochemical and Engineering Aspects, 658 , 130748.
Cho, D.-W., Yoon, K., Ahn, Y., Sun, Y., Tsang, D. C., Hou, D., Ok, Y. S., & Song, H. (2019). Fabrication and environmental applications of multifunctional mixed metal-biochar composites (MMBC) from red mud and lignin wastes. Journal of Hazardous Materials, 374 , 412–419.
Corlin, L., Rock, T., Cordova, J., Woodin, M., Durant, J. L., Gute, D. M., Ingram, J., & Brugge, D. (2016). Health effects and environmental justice concerns of exposure to uranium in drinking water. Current Environmental Health Reports, 3 , 434–442.
da Silva Veiga, P. A., Schultz, J., da Silva Matos, T. T., Fornari, M. R., Costa, T. G., Meurer, L., & Mangrich, A. S. (2020). Production of high-performance biochar using a simple and low-cost method: optimization of pyrolysis parameters and evaluation for water treatment. Journal of Analytical and Applied Pyrolysis, 148 , 104823.
Dutta, D. P., & Nath, S. (2018). Low cost synthesis of SiO 2 /C nanocomposite from corn cobs and its adsorption of uranium (VI), chromium (VI) and cationic dyes from wastewater. Journal of Molecular Liquids, 269 , 140–151.
Glaser, B., Lehmann, J., & Zech, W. (2002). Ameliorating physical and chemical properties of highly weathered soils in the tropics with charcoal–a review. Biology and Fertility of Soils, 35 , 219–230.
Guo, J., Yan, C., Luo, Z., Fang, H., Hu, S., & Cao, Y. (2019). Synthesis of a novel ternary HA/Fe-Mn oxides-loaded biochar composite and its application in cadmium (II) and arsenic (V) adsorption. Journal of Environmental Sciences, 85 , 168–176.
Hadjittofi, L., & Pashalidis, I. (2015). Uranium sorption from aqueous solutions by activated biochar fibres investigated by FTIR spectroscopy and batch experiments. Journal of Radioanalytical and Nuclear Chemistry, 304 , 897–904.
Huang, Y., Hu, Y., Chen, L., Yang, T., Huang, H., Shi, R., Lu, P., & Zhong, C. (2018). Selective biosorption of thorium (IV) from aqueous solutions by ginkgo leaf. PLoS One, 13 , e0193659.
Article Google Scholar
Jin, J., Li, S., Peng, X., Liu, W., Zhang, C., Yang, Y., Han, L., Du, Z., Sun, K., & Wang, X. (2018). HNO 3 modified biochars for uranium (VI) removal from aqueous solution. Bioresource Technology, 256 , 247–253.
Krachler, R., Krachler, R., Gülce, F., Keppler, B. K., & Wallner, G. (2018). Uranium concentrations in sediment pore waters of Lake Neusiedl, Austria. Science of the Total Environment, 633 , 981–988.
Kumar, M., Dutta, S., You, S., Luo, G., Zhang, S., Show, P. L., Sawarkar, A. D., Singh, L., & Tsang, D. C. (2021). A critical review on biochar for enhancing biogas production from anaerobic digestion of food waste and sludge. Journal of Cleaner Production, 305 , 127143.
Li, M., Liu, H., Chen, T., Dong, C., & Sun, Y. (2019). Synthesis of magnetic biochar composites for enhanced uranium (VI) adsorption. Science of the Total Environment, 651 , 1020–1028.
Li, N., Yin, M., Tsang, D. C., Yang, S., Liu, J., Li, X., Song, G., & Wang, J. (2019). Mechanisms of U (VI) removal by biochar derived from Ficus microcarpa aerial root: A comparison between raw and modified biochar. Science of the Total Environment, 697 , 134115.
Liao, J., Xiong, T., Ding, L., Zhang, Y., & Zhu, W. (2022). Effective separation of uranium (VI) from wastewater using a magnetic carbon as a recyclable adsorbent. Separation and Purification Technology, 282 , 120140.
Lin, X.-Q., Lü, Q.-F., Li, Q., Wu, M., & Liu, R. (2018). Fabrication of low-cost and ecofriendly porous biocarbon using konjaku flour as the raw material for high-performance supercapacitor application. ACS Omega, 3 , 13283–13289.
Lv, Z., Yang, S., Chen, L., Alsaedi, A., Hayat, T., & Chen, C. (2019). Nanoscale zero-valent iron/magnetite carbon composites for highly efficient immobilization of U (VI). Journal of Environmental Sciences, 76 , 377–387.
Mangeret, A., Blanchart, P., Alcalde, G., Amet, X., Cazala, C., & Gallerand, M.-O. (2018). An evidence of chemically and physically mediated migration of 238U and its daughter isotopes in the vicinity of a former uranium mine. Journal of Environmental Radioactivity, 195 , 67–71.
Mishra, A., Kumar, M., Bolan, N. S., Kapley, A., Kumar, R., & Singh, L. (2021). Multidimensional approaches of biogas production and up-gradation: Opportunities and challenges. Bioresource Technology, 338 , 125514.
Peng, P., Lang, Y.-H., & Wang, X.-M. (2016). Adsorption behavior and mechanism of pentachlorophenol on reed biochars: pH effect, pyrolysis temperature, hydrochloric acid treatment and isotherms. Ecological Engineering, 90 , 225–233.
Philippou, K., Anastopoulos, I., Dosche, C., & Pashalidis, I. (2019). Synthesis and characterization of a novel Fe 3 O 4 -loaded oxidized biochar from pine needles and its application for uranium removal. Kinetic, thermodynamic, and mechanistic analysis. Journal of Environmental Management, 252 , 109677.
Qiu, B., Tao, X., Wang, H., Li, W., Ding, X., & Chu, H. (2021). Biochar as a low-cost adsorbent for aqueous heavy metal removal: A review. Journal of Analytical and Applied Pyrolysis, 155 , 105081.
Safarik, I., & Safarikova, M. (2014). One-step magnetic modification of non-magnetic solid materials. International Journal of Materials Research, 105 , 104–107.
Sun, Y., Iris, K., Tsang, D. C., Cao, X., Lin, D., Wang, L., Graham, N. J., Alessi, D. S., Komárek, M., & Ok, Y. S. (2019). Multifunctional iron-biochar composites for the removal of potentially toxic elements, inherent cations, and hetero-chloride from hydraulic fracturing wastewater. Environment International, 124 , 521–532.
Tsouris, C. (2017). Uranium extraction: fuel from seawater. Nature Energy, 2 , 1–3.
Google Scholar
Uchimiya, M., Chang, S., & Klasson, K. T. (2011). Screening biochars for heavy metal retention in soil: role of oxygen functional groups. Journal of Hazardous Materials, 190 , 432–441.
Vithanage, M., Rajapaksha, A. U., Ahmad, M., Uchimiya, M., Dou, X., Alessi, D. S., & Ok, Y. S. (2015). Mechanisms of antimony adsorption onto soybean stover-derived biochar in aqueous solutions. Journal of Environmental Management, 151 , 443–449.
Wang, P., Wang, X., Yu, S., Zou, Y., Wang, J., Chen, Z., Alharbi, N. S., Alsaedi, A., Hayat, T., & Chen, Y. (2016). Silica coated Fe 3 O 4 magnetic nanospheres for high removal of organic pollutants from wastewater. Chemical Engineering Journal, 306 , 280–288.
Wang, W., Tang, B., Ju, B., Gao, Z., Xiu, J., & Zhang, S. (2017). Fe 3 O 4 -functionalized graphene nanosheet embedded phase change material composites: efficient magnetic-and sunlight-driven energy conversion and storage. Journal of Materials Chemistry A., 5 , 958–968.
Wang, X., Feng, J., Cai, Y., Fang, M., Kong, M., Alsaedi, A., Hayat, T., & Tan, X. (2020). Porous biochar modified with polyethyleneimine (PEI) for effective enrichment of U (VI) in aqueous solution. Science of the Total Environment, 708 , 134575.
Xue, Y., Gao, B., Yao, Y., Inyang, M., Zhang, M., Zimmerman, A. R., & Ro, K. S. (2012). Hydrogen peroxide modification enhances the ability of biochar (hydrochar) produced from hydrothermal carbonization of peanut hull to remove aqueous heavy metals: Batch and column tests. Chemical Engineering Journal, 200 , 673–680.
Ye, T., Huang, B., Wang, Y., Zhou, L., & Liu, Z. (2020). Rapid removal of uranium (VI) using functionalized luffa rattan biochar from aqueous solution. Colloids and Surfaces A: Physicochemical and Engineering Aspects, 606 , 125480.
Zhang, Z.-b., Cao, X.-h., Liang, P., & Liu, Y.-h. (2013). Adsorption of uranium from aqueous solution using biochar produced by hydrothermal carbonization. Journal of Radioanalytical and Nuclear Chemistry, 295 , 1201–1208.
Zhang, X., Yao, Z., Zhou, Y., Zhang, Z., Lu, G., & Jiang, Z. (2021). Theoretical guidance for the construction of electron-rich reaction microcenters on C–O–Fe bridges for enhanced Fenton-like degradation of tetracycline hydrochloride. Chemical Engineering Journal, 411 , 128535.
Zuo, X., Liu, Z., & Chen, M. (2016). Effect of H 2 O 2 concentrations on copper removal using the modified hydrothermal biochar. Bioresource Technology, 207 , 262–267.
Download references
Acknowledgments
This work was supported by the National Natural Science Foundation of China (42207306) , Natural Science Foundation of Chongqing, China (cstc2021jcyj-bshX0231) and Fund of Chongqing Engineering Research Center for Soil Contamination Control and Remediation (2022H001).
Author information
Authors and affiliations.
College of River and Ocean Engineering, Chongqing Jiaotong University, Chongqing, 400074, China
Zhenzhen Xu, Yangyang Zhang & Bo Zu
Dongfang Green Energy (Hebei) Co., Ltd., Hebei, 061000, China
Hangxiang Li
You can also search for this author in PubMed Google Scholar
Contributions
All authors contributed to the study conception and design. Material preparation, data collection and analysis were performed by Zhenzhen Xu and Hangxiang Li. The first draft of the manuscript was written by Zhenzhen Xu. The manuscript was revised by Yangyang Zhang and Bo Zu.
Corresponding authors
Correspondence to Yangyang Zhang or Bo Zu .
Ethics declarations
Conflict of interest.
On behalf of all authors, the corresponding author states that there is no conflict of interest.

Additional information
Publisher’s note.
Springer Nature remains neutral with regard to jurisdictional claims in published maps and institutional affiliations.
Rights and permissions
Springer Nature or its licensor (e.g. a society or other partner) holds exclusive rights to this article under a publishing agreement with the author(s) or other rightsholder(s); author self-archiving of the accepted manuscript version of this article is solely governed by the terms of such publishing agreement and applicable law.
Reprints and permissions
About this article
Xu, Z., Li, H., Zhang, Y. et al. Enhanced Adsorption of Uranium (VI) from Aqueous Solutions by Hydrogen Peroxide-Modified Magnetic Biochar: Performance Evaluation and Mechanistic Study. Water Air Soil Pollut 235 , 708 (2024). https://doi.org/10.1007/s11270-024-07517-3
Download citation
Received : 29 April 2024
Accepted : 14 September 2024
Published : 27 September 2024
DOI : https://doi.org/10.1007/s11270-024-07517-3
Share this article
Anyone you share the following link with will be able to read this content:
Sorry, a shareable link is not currently available for this article.
Provided by the Springer Nature SharedIt content-sharing initiative
- Hydrogen peroxide
- Find a journal
- Publish with us
- Track your research
Investigation: How Does Concentration Affect the Reaction Rates of Enzymes
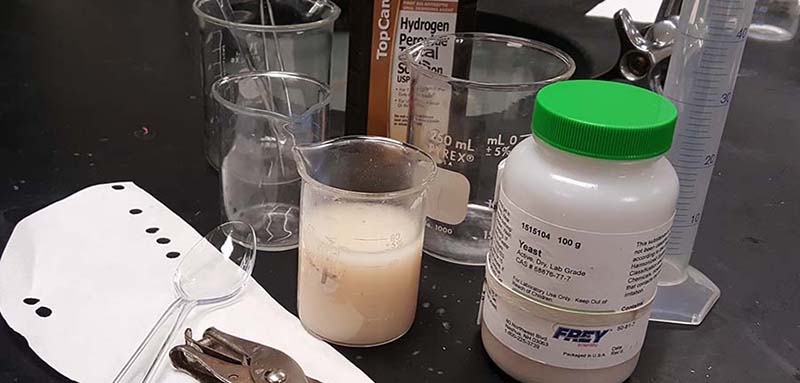
- to observe the catalytic action of yeast catalase on hydrogen peroxide
- to determine the effect of concentration on yeast catalase activity
- to determine the effect of temperature on yeast catalase activity
Background information:
Hydrogen peroxide (H 2 O 2 ) is a common but poisonous by-product of cellular metabolism, but H 2 O 2 does not accumulate in cells because it is decomposed to water and oxygen gas. The decomposition of the hydrogen peroxide is facilitated by catalase, an enzyme present in most cells.
The reaction is: 2H 2 O 2 → 2H 2 O + O 2
One molecule of catalase can catalyze the decomposition of approximately 4 x 10 7 molecules H 2 O 2 per second!
In this lab activity, you will be using yeast catalase to observe how increasing and decreasing the concentration of the enzyme and substrate can affect the reaction rate.
Hydrogen Peroxide (3%) | Multiple beakers or cups (100-300 ml) or test tubes Graduated cylinders or other measuring tools Filter paper and Hole punch | Forceps | Water | Stopwatch or timer | Active dry yeast
Create your stock catalase solution
1. Dissolve 1 tsp (2-4 grams) of yeast in 200 ml of warm water. 2. Mix well and let sit for about 3 minutes 3. Test the activity of your yeast by adding a few drops to 5 ml of H 2 O 2 - observe bubbles.
Observation of Catalase Activity
1. Pour 80 ml of H 2 O 2 into a small beaker or test tube. 2. Cut a filter paper disk using a hole punch and soak this in your stock catalase, blot on a paper towel. 3. Drop the disk into the H 2 O 2 .
Describe your observations.
If nothing happens (disk just floats) you may need to troubleshoot your experiment. Try stirring your catalase solution or dipping the disk in the H 2 O 2 to break the surface tension. If you are still not seeing anything happen, consult your instructor.
4. Perform this procedure again and record the time it takes for the disk to drop and then raise to the surface. Perform multiple trials to perfect your technique. Convert all readings to seconds to take an average.
Trial 1 | Trial 2 | Trial 3 | Average | |
Time |
Effect of Substrate Concentration
Your H 2 O 2 started at 3%. You will now dilute the peroxide in order to change its concentration.
1. Place 40 ml of H 2 O 2 into a new beaker. Add 40 ml of water. H 2 O 2 concentration = 1.5% 2. Place 20 ml of H 2 O 2 into a new beaker. Add 60 ml of water. H 2 O 2 concentration = .75% 3. Place 10 ml of H 2 O 2 into a new beaker. Add 70 ml of water. H 2 O 2 concentration = .375%
4. Perform the floating disk procedure for each concentration. You can copy your data for the 0.3% from the table above.
0.3% H O | 1.5% H O | 0.75% H O | 0.375% H O | |
Trial 1 | ||||
Trial 2 | ||||
Trial 3 | ||||
Average |
5. Create a graph that compares the averages for each concentration. Be sure to label the X and Y axis.
6. Use your graph and data to make a CLAIM that answers the question: How does decreasing the concentration of the substrate affect the enzyme reaction rate. This should be written in a complete sentence.
Effect of Enzyme Concentration
Your stock solution of catalase is your 100% solution. Create diluted solutions according to the ratios below and place each in small cups. These cups will be used to dip your filter paper disks.
1. 100% = 20 ml of catalase + 0 ml of water 2. 80 % = 16 ml of catalase + 4 ml of water 3. 60% = 12 ml of catalase + 8 ml of water 4. 40% = 8 ml of catalase + 12 ml of water 5. 20% = 4 ml of catalase + 16 ml of water.
6. Perform the floating disk procedure for each concentration. (We will not be taking averages this time.)
100% catalase | 80% catalase | 60% catalase | 40% catalase | 20% catalase | |
Time |
7. Use your graph and data to make a CLAIM that answers the question: How does decreasing the concentration of the enzyme affect the reaction rate.
8. Provide EVIDENCE for this claim by briefly summarizing your data or observations.
9. Consider how enzymes and substrates interact with each other. Suggest a REASON for your claim. This is where you consider what scientists understand about enzymes.
10. Which of the two variables (enzyme concentration or substrate concentration) seem to have the biggest affect on the reaction rate. How do you know?
Inquiry Version:
This lab can be a true inquiry lab after students complete the liver enzyme lab . In that case, students are not given these directions, just some tips on how to make a stock solution and how to perform dilutions. After seeing how to use the floating disk as a measure of reaction rate, students then design their own experiments to answer the experimental question.
Troubleshooting Tips
1. There are no bubbles or the disk will not float after sinking. - Check the hydrogen peroxide expiration date. The chemical will naturally break down into water over time and if exposed to light. Also, this may occur if the yeast hasn't really had time to start working. Use warm water, and you can make a stock solution the day before that will be ready to go, but do refrigerate over night.
2. The disk floats (will not sink). - This can occur if you aren't using filter paper or if the disk is just sitting on the surface of the water. Use forceps to break the surface tension instead of just dropping it onto the surface. This can also happen in later trials if the peroxide gets too much catalase in it from previous trials. The solution will just get "bubbly." Replace the solution with new.
3. Initial reaction is too fast and disk sinks very briefly and quickly rises to the surface. - Dilute the stock catalase solution.
Other Resources on Enzymes
Slides: Metabolism, Energy, and Enzymes | Guided Notes
Enzyme Practice - Label enzyme and substrate, idenfity types of feedback inhibition
Observe Catalase Activity in Yeast - create sodium alginate spheres to observe how catalase breaks down hydrogen peroxide
Enzyme Activity Using Toothpickase - simulate the activity of an enzyme by breaking toothpick
Remember Me
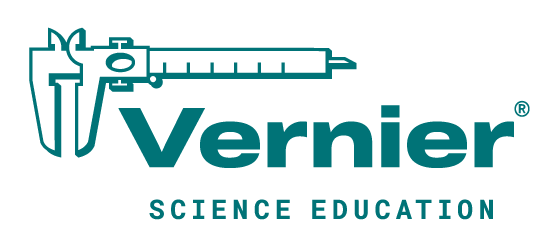
Shop Experiment Testing Catalase Activity (Gas Pressure) Experiments
Testing catalase activity (gas pressure).
Experiment #6B from Investigating Biology through Inquiry
Introduction
Many organisms can decompose hydrogen peroxide (H 2 O 2 ) enzymatically. Enzymes are globular proteins, responsible for most of the chemical activities of living organisms. They act as catalysts , substances that speed up chemical reactions without being destroyed or altered during the process. Enzymes are extremely efficient and may be used over and over again. One enzyme may catalyze thousands of reactions every second.
H2O2 is toxic to most living organisms. Many organisms are capable of enzymatically destroying the H 2 O 2 before it can do much damage. H 2 O 2 can be converted to oxygen and water, as follows:
Although this reaction occurs spontaneously, enzymes increase the rate considerably. At least two different enzymes are known to catalyze this reaction: catalase , found in animals and protists, and peroxidase , found in plants. A great deal can be learned about enzymes by studying the rates of enzyme-catalyzed reactions.
In this Preliminary Activity, you will use catalase in yeast to catalytically decompose hydrogen peroxide. You will use an O 2 Gas Sensor to determine the rate of catalase activity by measuring oxygen gas produced as H 2 O 2 is decomposed.
Before data collection begins, there is no product, and the pressure is the same as atmospheric pressure. Shortly after data collection begins, oxygen accumulates at a rather constant rate. The slope of the curve at this initial time is constant and is called the initial rate. In this investigation, we will refer to this as the rate of catalase activity. As the peroxide is decomposed, less of it is available to react and the O 2 is produced at lower rates. When no more peroxide is left, O 2 is no longer produced. When data collection is complete, you will perform a linear fit on the resultant graph to determine catalase activity.
After completing the Preliminary Activity, you will first use reference sources to find out more about catalase, enzymes, and enzyme activity before you choose and investigate a researchable question dealing with catalase activity. Some topics to consider in your reference search are:
- hydrogen peroxide
- collision theory
- reaction rate
Sensors and Equipment
This experiment features the following sensors and equipment. Additional equipment may be required.
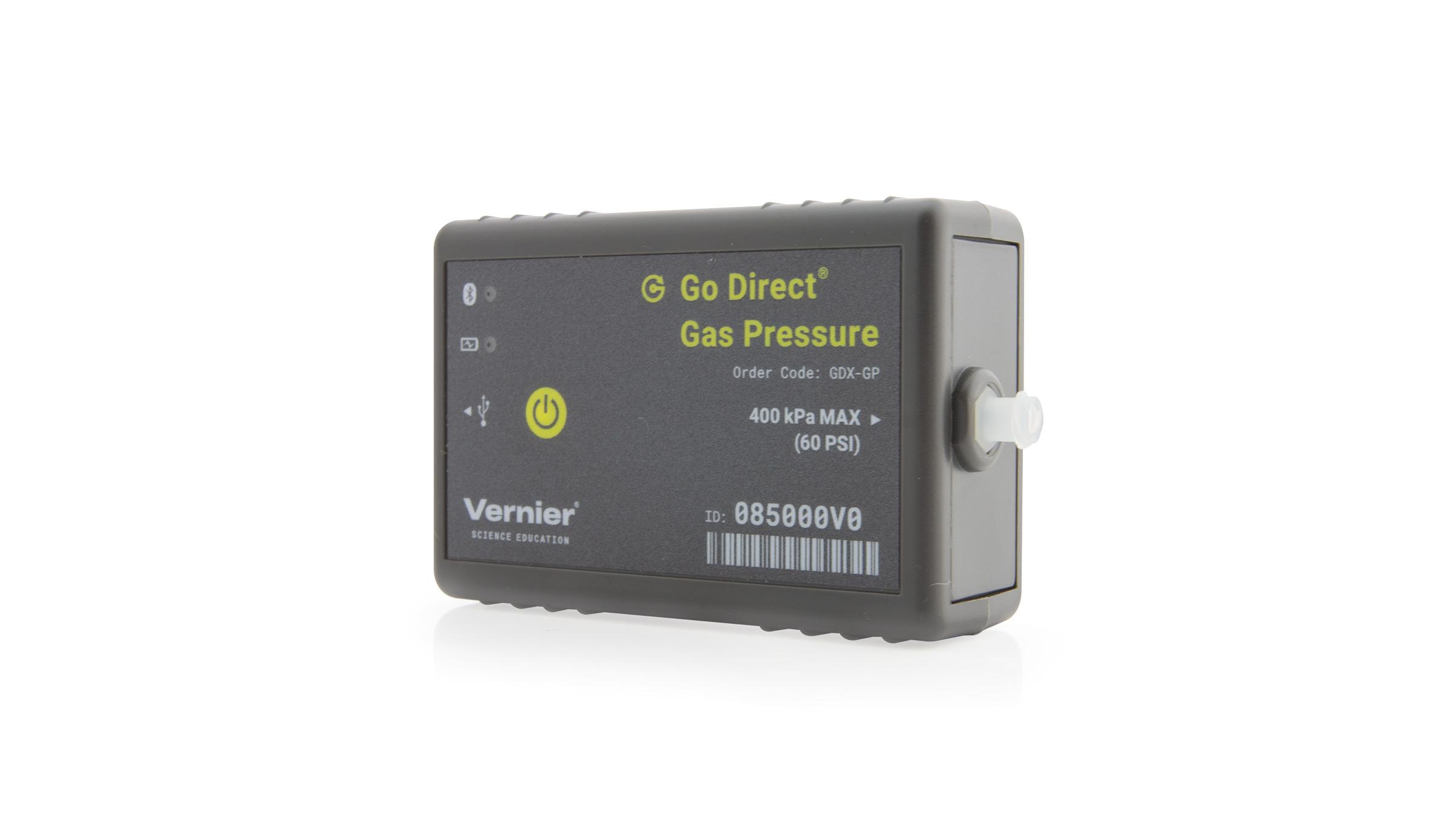
Ready to Experiment?
Ask an expert.
Get answers to your questions about how to teach this experiment with our support team.
- Call toll-free: 888-837-6437
- Chat with Us
- Email [email protected]
Purchase the Lab Book
This experiment is #6B of Investigating Biology through Inquiry . The experiment in the book includes student instructions as well as instructor information for set up, helpful hints, and sample graphs and data.
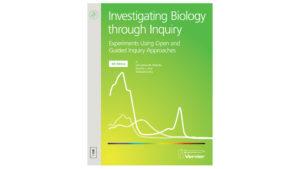
- Open access
- Published: 23 September 2024
It takes two peroxisome proliferator-activated receptors (PPAR-β/δ and PPAR-γ) to tango idiopathic pulmonary fibrosis
- Eistine Boateng 1 nAff8 ,
- Rocio Bonilla-Martinez 1 ,
- Barbara Ahlemeyer 1 ,
- Vannuruswamy Garikapati 1 , 6 nAff9 ,
- Mohammad Rashedul Alam 1 ,
- Omelyan Trompak 2 ,
- Gani Oruqaj 1 nAff10 ,
- Natalia El-Merhie 1 nAff11 ,
- Michael Seimetz 3 ,
- Clemens Ruppert 3 , 4 ,
- Andreas Günther 3 , 5 ,
- Bernhard Spengler 6 ,
- Srikanth Karnati 1 na1 nAff7 &
- Eveline Baumgart-Vogt 1 na1
Respiratory Research volume 25 , Article number: 345 ( 2024 ) Cite this article
390 Accesses
3 Altmetric
Metrics details
Idiopathic pulmonary fibrosis (IPF) is characterized by aberrant lung epithelial phenotypes, fibroblast activation, and increased extracellular matrix deposition. Transforming growth factor-beta (TGF-β)1-induced Smad signaling and downregulation of peroxisomal genes are involved in the pathogenesis and can be inhibited by peroxisome proliferator-activated receptor (PPAR)-α activation. However, the three PPARs, that is PPAR-α, PPAR-β/δ, and PPAR-γ, are known to interact in a complex crosstalk.
To mimic the pathogenesis of lung fibrosis, primary lung fibroblasts from control and IPF patients with comparable levels of all three PPARs were treated with TGF-β1 for 24 h, followed by the addition of PPAR ligands either alone or in combination for another 24 h. Fibrosis markers (intra- and extracellular collagen levels, expression and activity of matrix metalloproteinases) and peroxisomal biogenesis and metabolism (gene expression of peroxisomal biogenesis and matrix proteins, protein levels of PEX13 and catalase, targeted and untargeted lipidomic profiles) were analyzed after TGF-β1 treatment and the effects of the PPAR ligands were investigated.
TGF-β1 induced the expected phenotype; e.g. it increased the intra- and extracellular collagen levels and decreased peroxisomal biogenesis and metabolism. Agonists of different PPARs reversed TGF-β1-induced fibrosis even when given 24 h after TGF-β1. The effects included the reversals of (1) the increase in collagen production by repressing COL1A2 promoter activity (through PPAR-β/δ activation); (2) the reduced activity of matrix metalloproteinases (through PPAR-β/δ activation); (3) the decrease in peroxisomal biogenesis and lipid metabolism (through PPAR-γ activation); and (4) the decrease in catalase protein levels in control (through PPAR-γ activation) and IPF (through a combined activation of PPAR-β/δ and PPAR-γ) fibroblasts. Further experiments to explore the role of catalase showed that an overexpression of catalase protein reduced collagen production. Additionally, the beneficial effect of PPAR-γ but not of PPAR-β/δ activation on collagen synthesis depended on catalase activity and was thus redox-sensitive.
Our data provide evidence that IPF patients may benefit from a combined activation of PPAR-β/δ and PPAR-γ.
IPF is a severe restrictive interstitial lung disease with patient median survival of 2.5–3.5 years [ 1 ]. Concerning the pathogenesis of IPF, it is being discussed that an excessive injury response results in persistent overproduction of extracellular matrix (ECM) components by activated and proliferating fibroblasts. In addition, oxidative stress remains a major mechanism associated with the progression of this disease [ 2 ]. Today, only limited treatment options for IPF are available. Evidence-based recommendations for the pharmacological management of the disease are the tyrosine kinase inhibitor nintedanib [ 3 , 4 ] and pirfenidone [ 4 , 5 ], an inhibitor of TGF-β1-stimulated collagen synthesis. Both drugs increase quality of life, attenuate symptoms and slow down IPF progression, but only nintedanib influences mortality. Some of the novel medications targeted pentraxin (involved in endogenous tissue repair), lysophosphatidic acid, or connective tissue growth factor (mediates TGF-β1 downstream signaling), but failed the clinical endpoints [ 6 , 7 ]. Other substances in the pipeline are nerandomilast (a tyrosine kinase inhibitor) which successfully completed phase II clinical trials [ 8 ] and inhaled treprostinil, a prostacyclin analogue. Treprostinil showed beneficial effects in the initial INCREASE trial [ 9 ] and ongoing TETON study [ 10 ] and has meanwhile been approved for the therapy of WHO group 1 pulmonary hypertension with an additional positive impact in IPF. Nevertheless, extensive research is still required to develop new therapeutic modalities.
To find therapeutic interventions for IPF, several studies explored the anti-fibrotic potentials of natural and synthetic PPAR ligands. For example, PPAR-α activation was demonstrated to attenuate fibrosis in the liver [ 11 ], heart [ 12 ] and lung [ 13 , 14 ], while PPAR-β agonists exhibited anti-proliferative effects [ 15 ], but increased the secretion of TGF-β1 and ECM [ 16 ]. Ligands of PPAR-γ are most promising [ 17 , 18 , 19 , 20 ] and were thought to inhibit fibroblast trans-differentiation [ 21 , 22 ] and to strengthen the anti-oxidative defense system [ 23 ]. In addition, pan-PPAR agonists, such as lanifibranor [ 24 ] and IVA337 [ 25 ] attenuated fibrosis. In all these studies, however, the anti-fibrotic mechanism of PPAR agonists remained unclear and was supposed to be mainly due to their anti-inflammatory activities [ 26 ]. Another drawback was the time schedule of the drug treatment. Typically, drugs were added before or together with TGF-β1, but these approaches do not reflect the patient situation where drugs can be given only after the diagnosis of the disease, years after its initiation. In two studies, PPAR-γ agonists were applied after bleomyin-induced lung injury in the mouse. Zeng et al. [ 27 ] added the PPARγ ligand asarinin 15–28 days after bleomycin administration, which reduced the severity of fibrosis. Speca et al. [ 22 ] applied GED-0507, a PPARγ modulator with strong anti-inflammatory effects, to mice on day 14 after bleomycin administration and reported resolution of fibrosis with 50% mortality rate. This post-treatment schedule reduced collagen deposition, but to a lesser extent than in the prevention approach used in the same study. Thus, we thought that a post-treatment with a combination of PPAR ligands may further increase the anti-fibrotic effect. Moreover, we aimed to use a human model and human cultured fibroblasts as the latter in vitro model better guaranties the drug availability and allows a selective (biochemical) analysis of changes in fibroblasts, the main players in fibrosis.
In this study, we investigated whether activation of each of the three PPARs alone or in various combinations influenced collagen synthesis and release of lung fibroblasts from control and IPF patients when given 24 h after TGF-β1, the endogenous stimulator of fibrosis. Moreover, we attempted to explore the mechanism of the anti-fibrotic effect of PPAR agonists by analyzing changes in members of matrix metalloproteinases (MMPs) [ 28 ], biogenesis and metabolism of peroxisomes [ 13 , 14 ], and the protein level and activity of catalase, the major anti-oxidative enzyme in peroxisomes [ 29 ] with the highest turnover numbers of all enzymes [ 30 ].
Study approval
Biospecimen collection (i.e. lung tissues and fibroblasts from organ donors) was approved by the Ethics Committee of the Justus Liebig University Giessen (Az58/15 and Az111/08, JLU).
Cell culture and drug treatment
Lung fibroblasts from control and IPF patients (Additional file: Table S1) and catalase-deficient fibroblast cell lines were cultured in Dulbecco´s Modified Eagle Medium (DMEM) with penicillin/streptomycin or puromycin, respectively. For the experiments, cells were serum-starved for 3 h, stimulated with vehicle or rhTGF-β1 for 24 h (except for Figs. 2 B, C, E, 3 B), followed by the addition of vehicle or drugs either alone or in combinations for another 24 h.
Knockdown of catalase in human lung fibroblasts
Knockdown of catalase was done with CAT siRNA using ScreenFectA transfection reagent. Stable catalase knockdown was achieved by transduction with pGIPZ-shCatalase and pGIPZ-non-silencing control lentivirus vectors as described earlier [ 31 ].
Overexpression of catalase in human lung fibroblasts
Transfection with catalase overexpression plasmid (pGL 4.14- Catalase ) and promoter reporter plasmids COL1A2 -luc and PPAR response element ( PPRE) -luc were done as described earlier [ 13 , 32 ]. Data from pRL-SV40 vector served to normalize results of the luciferase reporter plasmid.
Human TGF-β1 immunoassay and sircol collagen assay
The collected culture media of control and IPF fibroblasts were used for Sircol collagen assays and TGF-β1 ELISA assay according to the manufacturers´ instructions.
Measurements of catalase activity, hydrogen peroxide (H 2 O 2 ) production and cell proliferation
Determination of catalase activity with a redox dye assay kit based on the degradation of H 2 O 2 . H 2 O 2 produced by cultured cells was quantified using a fluorometric detection kit. The incorporation of BrdU into proliferating cells was detected with an ELISA kit. For all the aforementioned kits, we followed the manufacturers´ instructions.
Western blotting
Proteins of total cell lysates were separated on 10% SDS-PAGE gels and blotted on polyvinylidene difluoride membranes. Specific proteins were detected using primary and horseradish peroxidase (HRP)-labelled secondary antibodies followed by chemiluminescent detection of the HRP substrate. ImageJ was used for semi-quantitative analysis of signal intensities.
Immunofluorescence staining
Thin sections of paraffin-embedded lung tissues were incubated with primary and secondary fluorophore-labelled antibodies. Immunofluorescence images were acquired by confocal laser scanning microscopy.
Isolation of total RNA and RT-qPCR
Total RNA was isolated using RNAzol and mRNA levels were analyzed by RT-qPCR.
Targeted quantification of fatty acids
Arachidonic acid (AA), docosahexaenoic acid (DHA), and eicosapentaenoic acid (EPA) were analyzed in the culture medium by solid phase extraction and a targeted liquid chromatography tandem mass spectrometry (LC–MS/MS) approach as described previously [ 32 ].
Untargeted lipidomics
Lipids were extracted from cell lysates using a biphasic methyl- tert -butyl ether (MTBE) extraction protocol [ 33 ] and analyzed using an untargeted LC–MS/MS method as described previously [ 34 ].
Analysis was done using GraphPad Prism software. Data were expressed as means ± SEM. For comparisons between two groups, the F-test was applied to compare their variances followed by Mann–Whitney U test (unequal variances) or unpaired t -test (equal variances). For multiple comparisons, ANOVA was used with post-hoc Tukey´s multiple comparisons test. P values < 0.05 were considered as statistically significant.
Characterization of the fibrosis markers COL1 and α-SMA, as well as of PPARs in lung tissues and cultured fibroblasts from control and IPF patients
The fibrosis marker collagen type I (COL1) and myofibroblast marker α-smooth muscle actin (α-SMA) were first assessed in lung biopsy samples from control and IPF patients. Lung tissues from IPF patients showed comparatively higher levels of COL1 and α-SMA than those from control subjects (Fig. 1 A). Although increased mRNA levels of COL1A1 and ACTA2 were detected in cultured lung fibroblasts from IPF compared to control patients (Additional file: Fig. S1A, B), their protein levels and that of transforming growth factor-beta receptor 1 (TGFBR1) were higher in most cases in fibroblasts from control compared to IPF patients (Additional file: Fig. S1C, Table 1 ). Although unexpected at first glance, it is noteworthy that IPF lung tissue contains a much higher number of fibroblasts than controls. Probably, the higher number of fibroblasts in the lungs of IPF patients and to a minor extent their individual properties contribute to the differences in tissue pathology. Moreover, the reduced level of TGFBR1 in IPF fibroblasts suggests that they are less sensitive to TGF-β1 presumably due to their chronic exposure to the cytokine in vivo. Accordingly, analysis of extracellular collagen revealed no significant difference between control and IPF fibroblasts (Fig. 1 B, Additional file: Fig. S1D). IPF is characterized by elevated levels of TGF-β1 mRNA and protein in the lung tissues of patients [ 35 , 36 ]. Interestingly, the amount of released active TGF-β1 was higher in the culture media from control than IPF fibroblasts (Fig. 1 C). We demonstrated an anti-fibrotic role of peroxisomes in the progression of IPF via PPAR-α signaling [ 13 , 14 ]. Since all three PPARs crosstalk with each other [ 37 ], we next analyzed their protein levels in fibroblasts from control and IPF patients at basal conditions (no treatment in vitro). Collectively, IPF fibroblasts showed increased mRNA and protein levels of PPAR-α, but not of the ones of PPAR-β/δ and PPAR-γ compared to control fibroblasts (Fig. 1 D, Table 1 , Additional file: Fig. S1E).
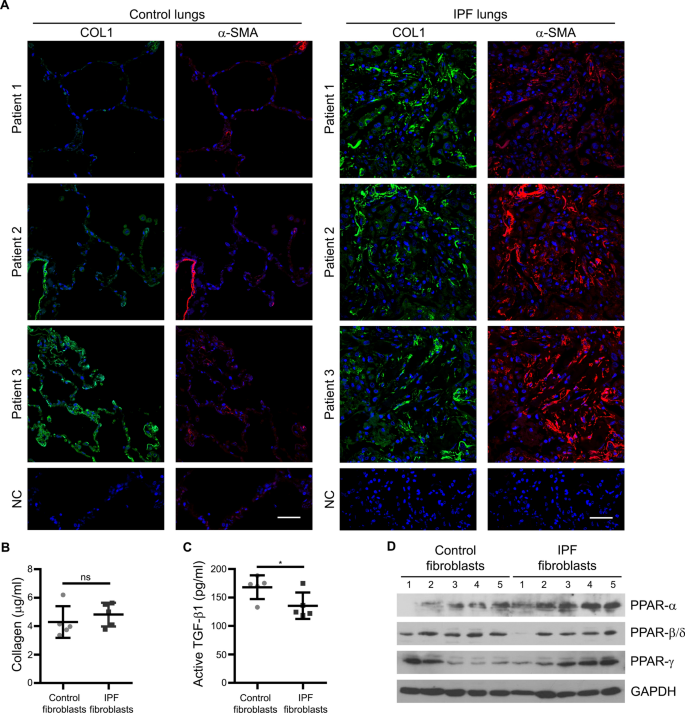
Characterization of the fibrosis markers COL1 and α-SMA, and PPARs in lung tissue and cultured fibroblasts from control and IPF patients. A Lung tissue sections from control (left side) and IPF (right side) patients were incubated with antibodies to detect collagen (COL1, green) and α-SMA (red), and counterstained with DAPI (blue). Negative controls (NC) were done by omitting the primary antibody . B There was no difference in the release of collagen between fibroblasts from control and IPF patients. The release of collagen into culture media was measured using Sircol assay. Data represent 5 control and 5 IPF patients across six independent fibroblast cultures. C The release of active TGF-β1 is higher in control than in IPF fibroblasts. The amount of active human TGF-β1 was analyzed in the culture media of fibroblasts from 5 controls and 7 IPF patients by ELISA. D The protein levels of PPAR-α were higher in IPF compared to control fibroblasts, whereas there was no difference with regard to PPAR-β/δ and PPAR-γ. Cultured fibroblasts from 5 control and 7 IPF patients were collected and their protein levels were analyzed by Western blot analysis with GAPDH as reference protein

TGF-β1 induced a fibrotic response in fibroblasts from control and IPF patients. A TGF-β1 induced proliferation in control and IPF fibroblasts. Fibroblasts were serum-starved for 3 h and then incubated for 24 h with vehicle or TGF-β1. Thereafter, proliferation was analyzed using BrdU cell proliferation assay. B Treatment with different concentrations of TGF-β1 showed no difference between control and IPF fibroblasts with regard to the release of collagen into culture media. Control and IPF fibroblasts were serum-starved for 3 h and then treated with vehicle (Control) or 2.5, 5, 10 and 20 ng/ml TGF-β1 for 24 h. Cell culture media were collected and extracellular collagen was analyzed using Sircol assay. C , D TGF-β1 increased the level of intracellular COL1 in control and IPF fibroblasts in a time-dependent manner. Control and IPF fibroblasts were serum-starved for 3 h and then treated with vehicle or 5 ng/ml TGF-β1 for 12, 24, 36 and 48 h. Cell lysates were used to detect COL1 and α-SMA by Western blot analysis using GAPDH as reference protein ( C ). Data for a time period of 24 h from 5 control (patients A–E) and 5 IPF (patients F–J) patients is shown in ( D ). E TGF-β1 increased the protein level of PPAR-β/δ, whereas the ones of the other PPARs remained unchanged. Control and IPF fibroblasts were treated for 24, 48 and 72 h with TGF-β1 (5 ng/ml) or vehicle. Cell lysates were used for Western blot analysis of the PPARs using GAPDH as reference protein
Activation of PPAR-β/δ induced anti-fibrotic responses in TGF-β1-stimulated fibroblasts from control and IPF patients
As already noted, the number of fibroblasts in the lungs of IPF patients might be crucial for the disease progression. To confirm this, we analyzed the proliferation of vehicle- and TGF-β1-treated control and IPF fibroblasts since the cytokine was used to mimic part of the disease condition in vitro. As expected, TGF-β1 stimulated cell proliferation in control and IPF fibroblasts (Fig. 2 A). Next, we analyzed time-dependent changes in α-SMA and COL1 protein levels of control and IPF fibroblasts treated with TGF-β1. Control and IPF fibroblasts did not show differences after stimulation with different concentrations of TGF-β1 (2.5–20 ng/ml; Fig. 2 B) in the extracellular collagen released into the culture media. Though 2.5 ng/ml of TGF-β1 was already sufficient to reach the maximal effect for collagen values 24 h after treatment (Fig. 2 B), 5 ng/ml TGF-β1 was used to obtain maximal effects in all following experiments with distinct parameters. TGF-β1 increased intracellular COL1 and α-SMA protein levels from 12 to 48 h in control fibroblasts and from 24 h up to 48 h in IPF fibroblasts (Fig. 2 C). Moreover, the treatment with TGFβ-1 for 24 h in control and IPF fibroblasts from 10 different patients showed a homogenous and stable increase in the protein levels of COL1, but an inconsistent reaction in the case of α-SMA (Fig. 2 D, Table 1 ). To investigate the role of peroxisomes in IPF, their proliferation was induced using different PPAR ligands. Interestingly, TGF-β1 upregulated the protein level of PPAR-β/δ especially after 48 h of treatment (Fig. 2 E). Following 24 h TGF-β1 stimulation, treatment with PPAR-β/δ agonist alone or in combination with the two other members of the PPAR protein family inhibited the TGF-β1-mediated increase in COL1 and—to a lesser extent—α-SMA protein levels in control and IPF fibroblasts (Fig. 3 A). As already noted, anti-fibrotic properties of PPAR-γ have been reported in the past. In our study, the post-treatment with a PPAR-β/δ agonist (GW0742) alone or combined with a PPAR-γ agonist (rosiglitazone) strongly decreased the amount of TGF-β1-mediated increase in intracellular COL1 (Fig. 3 A–C) by affecting COL1A2 promotor activity (Fig. 3 D) as well as extracellular collagen (Fig. 3 E) in both, fibroblasts from control and IPF patients. Lesser effects were observed in the case of activation of PPAR-γ alone (Fig. 3 A, C, E). The decrease in the amount of COL1 as a result of the dual treatment of PPAR-β/δ and PPAR-γ agonists was stable over time (Fig. 3 B) and between patients (Fig. 3 C). Furthermore, the anti-fibrotic effects of a combined activation of PPAR-β/δ and PPAR-γ were blocked in the presence of PPAR-β/δ (GSK0660) and PPAR-γ (GW9662) antagonists (Fig. 3 F). In addition, we thought to use the compound STK 648389 (ZINC ID: 31,775,965), a putative dual agonist for PPAR-β/δ and PPAR-γ. However, analysis of the STK 648389 for its effect on collagen showed adverse effects and even increased extracellular collagen levels released by control and IPF fibroblasts after TGF-β1 exposure (Additional file: Fig. S2). Altogether, these findings suggest that although TGF-β1 increases the PPAR-β/δ protein as a protective adaptive mechanism, endogenous PPAR-β/δ activating ligands are probably diminished to prevent fibrosis in patients.

Activation of PPAR-β/δ induced anti-fibrotic responses in TGF-β1-stimulated fibroblasts. A – C , E Control and IPF fibroblasts were serum-starved for 3 h, treated with TGF-β1 (5 ng/ml) for 24 h, followed by the addition of the PPAR-α agonist WY14643 (100 μM, α; A ), PPAR-β/δ agonist GW0742 (10 μM, β; A–C , E ), and PPAR-γ agonist rosiglitazone (10 μM, γ; A – C , E ) either for 24 h ( A , C , E) or different time periods (12, 24, 36 and 48 h; B ). A PPAR-β/δ activation reversed TGF-β1-induced increase in COL1. Cell lysates were used to detect COL1 and α-SMA by Western blot analysis using GAPDH as reference protein. B , C Reverse of fibrosis phenotype by PPAR-β/δ and PPAR-γ activation was stable for up to 48 h. Cell lysates at 12 to 24 h ( B ) and 48 h from two other control and IPF patients ( C ) were used for Western blot analysis using β-actin (β-ACTIN) as reference protein. D Combined activation of PPAR-β/δ and PPAR-γ abolished TGF-β1-induced increase in COL1A2 promoter activity. IPF fibroblasts were transfected with a plasmid containing the luciferase firefly reporter gene adjacent to COL1A2 promoter and Renilla luciferase as second reporter for normalization. At 72 h after transfection, cells were treated with vehicle (Vector) or TGF-β1 (5 ng/ml) for 24 h followed by the addition of the PPAR-β/δ agonist GW0742 (10 μM, β) combined with the PPAR-γ agonist rosiglitazone (10 μM, γ) or vehicle for another 24 h. Cells were lysed and collected for dual luciferase activity measurements. E Ligand activation of PPAR-β/δ together with PPAR-γ strongly decreased the release of collagen produced by TGF-β1-stimulation in control and IPF fibroblasts. Culture media were collected and extracellular collagen was analyzed using Sircol assay. F Combined activation of PPAR-β/δ and PPAR-γ decreased TGF-β1-stimulated release of collagen by control and IPF fibroblasts—this effect was blocked using the respective antagonists. Cells were serum-starved for 3 h, stimulated with vehicle (Control) or TGF-β1 (5 ng/ml) for 24 h, followed by the addition of the PPAR-β/δ agonist GW0742 (10 μM, β) and PPAR-γ agonist rosiglitazone (10 μM, γ) either combined with vehicle or the PPAR-β/δ antagonist GSK0660 (10 nM, β ant) and PPAR-γ antagonist GW9662 (10 μM, γ ant) for another 24 h. Culture media were collected and extracellular collagen was analyzed by Sircol assay
PPAR-β/δ triggers anti-fibrotic responses by activating MMP-1 in control and IPF fibroblasts
Extracellular collagen is degraded by proteinases, e.g. MMPs. The mRNA levels of selected MMPs in control fibroblasts at basal condition (without treatment) were measured, showing the highest value for MMP1 in comparison to the lower mRNA values for MMP2 , MMP3 , MMP10 , and MMP16 (Fig. 4 A). Interestingly, the mRNA level of MMP7 which is associated with disease severity [ 28 ] was below detectable levels in our samples of control and IPF fibroblasts (ct values > 35). Comparing the mRNA levels between control and IPF fibroblasts, no differences were observed in the case of MMP1 and MMP16 (Fig. 4 B, F), but higher levels were found for MMP2 , MMP3 and MMP10 (Fig. 4 C–E). Individual mRNA values for MMP1 , but also for MMP3 and MMP10 , varied strongly within the IPF sample group (Fig. 4 B, D, E). Due to the much higher mRNA levels for MMP1 compared to the other MMPs (Fig. 4 A), we analyzed MMP-1 protein as the dominant enzyme for collagen degradation in subsequent experiments. As expected, the protein level of active MMP-1 was reduced by TGF-β1 and restored in the presence of PPAR-β/δ agonist alone or in combination with PPAR-α or PPAR-γ agonists (Fig. 4 G). This suggests that PPAR-β/δ might be a key regulator of the protein level of active MMP-1. Therefore, we analyzed the effect of the PPAR-β/δ agonist in TGF-β1-stimulated fibroblasts at the mRNA levels of all detectable MMPs . The mRNA levels of MMP1 in IPF fibroblast were increased (> fivefold) by the PPAR-β/δ agonist in comparison to TGF-β1 stimulation alone (Fig. 4 H). The MMP16 mRNA levels were elevated > fivefold in both types of fibroblasts and that of MMP10 about threefold in control fibroblasts only (Additional file: Fig. S3). To explore the anti-fibrotic potential of increased levels of MMP s , we used a broad-spectrum inhibitor for MMPs, primarily influencing the amount of extracellular collagen. Simultaneous treatment with the MMP inhibitor and PPAR-β/δ agonist after TGF-β1 stimulation increased extracellular collagen in the culture media released by control fibroblasts, but not in the case of IPF fibroblasts (Fig. 4 I). Since the MMP inhibitor only partly blocked the effect of the PPAR-β/δ agonist, we speculate that activated PPAR-β/δ also regulates other proteins involved in fibrosis attenuation.
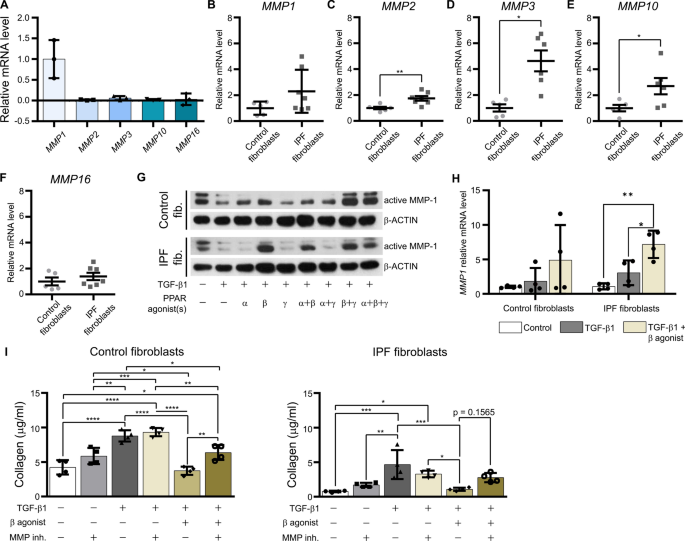
PPAR-β/δ triggers anti-fibrotic responses by activating MMP-1 in control and IPF fibroblasts. A The transcript of MMP1 is the highest among the different MMPs in control fibroblasts. Analysis of MMP1, MMP2, MMP3, MMP10 and MMP16 of control fibroblasts was done using isolated total RNA and RT-qPCR with HPRT1 as reference gene. B – F Comparative gene expression profile of MMPs was done by RT-qPCR with HPRT1 as reference gene. G PPAR-β/δ attenuated TGF-β1-induced decrease in the amount of active MMP-1. Control and IPF fibroblasts were serum-starved for 3 h, treated with vehicle or TGF-β1 (5 ng/ml) for 24 h, followed by the addition of the PPAR-α agonist WY14643 (100 μM, α), PPAR-β/δ agonist GW0742 (10 μM, β), and PPAR-γ agonist rosiglitazone (10 μM, γ) as well as various combinations thereof for another 24 h. Cell lysates were used to detect active MMP-1 by Western blot analysis using β-actin (β-ACTIN) as reference protein. H Ligand activation of PPAR-β/δ strongly increased the mRNA level of MMP1 in TGF-β1-treated control and IPF fibroblasts. Cells were serum-starved, treated with vehicle (Control) or TGF-β1 (5 ng/ml) for 24 h followed by the addition of the PPAR-β/δ agonist GW0742 (10 μM, β) or vehicle for another 24 h. The mRNA levels were measured by RT-qPCR with HPRT1 as reference gene. I Inhibition of MMPs increased TGF-β1-induced release of collagen. Control and IPF fibroblasts were serum-starved for 3 h, treated with vehicle or TGF-β1 (5 ng/ml) for 24 h, followed by the addition of the PPAR-β/δ agonist GW0742 (10 μM, β) and MMP inhibitor (MMP inh., 4-aminobenzoyl-Gly-Pro-D-Leu-D-Ala hydroxamic acid, 20 μM) for another 24 h. The release of collagen into the culture media was measured by Sircol assay
Activation of PPAR-β/δ and PPAR-γ in TGF-β1-treated fibroblasts increased peroxisomal biogenesis and lipid metabolism, and the inhibited fibrotic response
Previously, we showed that pretreatment with PPAR-α agonists could inhibit fibrosis phenotypes [ 13 , 14 ]. In the present study, we treated control and IPF fibroblasts with TGF-β1 before the addition of agonists of all three PPARs, an experimental setup that more accurately recapitulates the clinical setting. We first investigated the mRNA levels of several peroxisomal genes involved in the organelle biogenesis ( PEX13, PEX14 ), plasmalogen synthesis ( AGPS, GNPAT ), and fatty acid β-oxidation ( ACOX1, ACAA1 ) in control and IPF fibroblasts. The mRNA levels of PEX13 , ACOX1 and AGPS were higher in IPF compared to control fibroblasts, whereas those of PEX14, ACAA1 and GNPAT were not significantly different (Additional file: Fig. S4A–F). Next, we stimulated peroxisomal proliferation with different PPAR agonists (alone or in combination) in TGF-β1-treated control and IPF fibroblasts. Combined activation of PPAR-β/δ and PPAR-γ increased mRNA (Additional file: Fig. S4G) and protein levels (Fig. 5 A) of PEX13 in TGF-β1-stimulated control and IPF fibroblasts compared to TGF-β1 treatment only. Since the combined activation of PPAR-β/δ and PPAR-γ reversed the TGF-β1-induced trans-differentiation of fibroblasts into myofibroblasts (as indicated by changes in the level of α-SMA, Fig. 3 A–C), decreased the protein level of COL1 (Fig. 3 A–C) and increased PEX13 (Fig. 5 A), we focused on these two PPAR agonists in the following experiments. First, the intracellular lipidomic profile was assessed in control and IPF fibroblasts to ascertain possible differences in the lipid metabolism under basal conditions and after treatments with TGF-β1 alone and PPAR-β/δ and PPAR-γ agonists. In total, 1003 lipid ion species covering 5 major lipid categories (glycerophospholipids, sphingolipids, glycerolipids, fatty acyls, and sterols) belonging to 22 lipid classes were identified based on high mass accuracy (5 ppm) and their fragmentation patterns (Additional file: Fig. S5A). Basal levels of all classes of lipids analyzed were lower in IPF fibroblasts except for the triglycerides (TG; Fig. 5 B). TGF-β1 decreased the levels of phosphatidylcholine (PC), phosphatidylethanolamine (PE), sphingomyelin (SM) and TG in IPF fibroblasts. The levels of PC, SM and TG were partially restored by a post-treatment with PPAR-β/δ and PPAR-γ agonists (Fig. 5 B). Furthermore, activation of PPAR-β/δ and PPAR-γ strongly increased the synthesis of peroxisome-derived AA, DHA, and EPA (Fig. 5 C), which are all endogenous activators of PPARs. In the absence of TGF-β1, PPAR agonists either increased or decreased the levels of PC in control and IPF fibroblasts (Additional file: Fig. S5B) and increased the levels of AA, DHA and EPA with PPAR-γ exhibiting the strongest effect on DHA (Additional file: Fig. S5C). This suggests that, the PPAR-γ agonist was the driving factor for the increase and release of AA, DHA and EPA in fibroblasts treated with TGF-β1 followed by combined PPAR-β/δ and PPAR-γ agonists treatment (Fig. 5 C). Collectively, activation of PPAR-β/δ and PPAR-γ potentially regulates the fibrosis phenotype by modulating peroxisomal lipid metabolism, but differently in control and IPF fibroblasts.
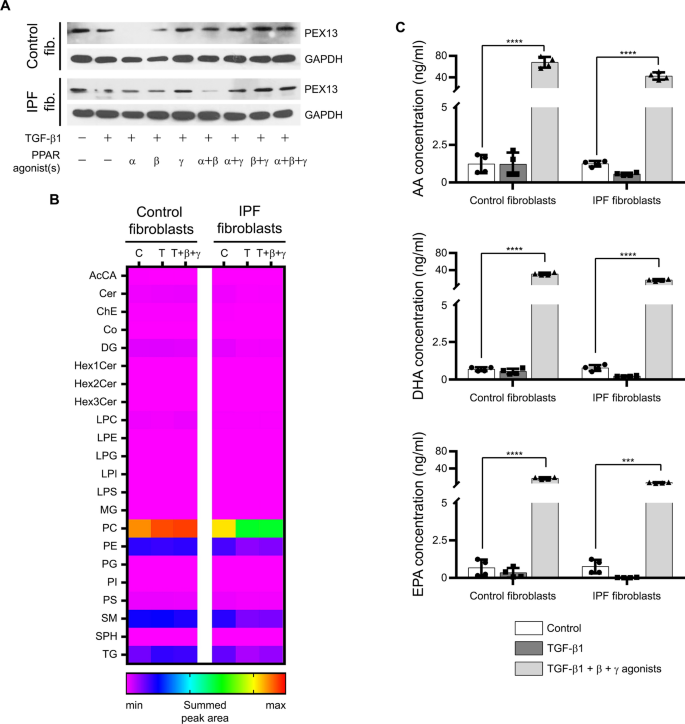
Activation of PPAR-β/δ and PPAR-γ in TGF-β1-treated fibroblasts increased peroxisomal biogenesis and lipid metabolism. A - C Control and IPF fibroblasts were serum-starved for 3 h, treated with vehicle or TGF-β1 (5 ng/ml) for 24 h, followed by the addition of the PPAR-α agonist WY14643 (100 μM, α; A ), PPAR-β/δ agonist GW0742 (10 μM, β; A – C ), and PPAR-γ agonist rosiglitazone (10 μM, γ; A-C ) as well as various combinations thereof for another 24 h. A Activation of PPAR-β/δ and PPAR-γ reversed TGF-β1-induced decrease in the protein levels of the peroxisomal biogenesis protein PEX13. Cell lysates were used for Western blot analysis of PEX13 using GAPDH as reference protein. B Heatmap of the lipidomic profile of control and IPF fibroblasts. Cells were collected in PBS for lipid analysis using LC–MS/MS. C Activation of PPAR-β/δ and PPAR-γ increased the synthesis of endogenous activators of these receptors in line with a positive feedback loop. Fibroblasts from control and IPF patients were serum-starved for 3 h, treated with vehicle (Control) or TGF-β1 (5 ng/ml) for 24 h, followed by the addition of vehicle or the PPAR-β/δ agonist GW0742 (10 μM, β) combined with the PPAR-γ agonist rosiglitazone (10 μM, γ) for another 24 h. The releases of AA, DHA, and EPA were analyzed in the culture media by LC–MS/MS
Activation of PPAR-β/δ in combination with PPAR-γ restored TGF-β1-induced decrease in catalase mRNA and protein levels
Though not significant, TGF-β1 decreased CAT mRNA level in control and IPF fibroblasts, which was restored by the combined activation of PPAR-β/δ and PPAR-γ (Additional file: Fig. S4G). We therefore speculated that this anti-oxidative enzyme might be involved in regulation of fibrogenesis. We first analyzed catalase and glutathione peroxidase (GPX)1/2 in human lung tissue samples. The protein level of catalase was markedly decreased in alveolar epithelial type II cells in the lungs of IPF compared to control patients (Fig. 6 A), whereas that of GPX1/2 was increased (Fig. 6 B), probably to compensate catalase deficiency. Moreover, we detected a gradual decrease in catalase protein level in mouse lungs after bleomycin-induced fibrosis, remarkably from day 14 after treatment (Additional file: Fig. S6A). When we analyzed the fibroblasts from control and IPF patients, we found no differences in the mRNA levels of CAT and GPX1/2 (Additional file: Fig. S6B, C). Protein level of catalase was lower in IPF compared to control fibroblasts (isolated from 5 patients each, Fig. 6 C, Table 1 ). Apart from catalase and GPX1/2, peroxiredoxins (PRDXs) were measured as they also support the anti-oxidant defense system. The mRNA levels of different peroxiredoxin family members varied strongly (Additional file: Fig. S6D) with PRDX6 showing the highest and PRXD2 and PRXD3 the lowest gene expression levels. Only the mRNA levels of PRDX4 and PRDX6 were significantly higher in IPF compared to control fibroblasts (Additional file: Fig. S6E–J). To confirm the regulatory effects of TGF-β1 on catalase, we treated control and IPF fibroblasts with TGF-β1 at various concentrations. Increasing concentrations of TGF-β1 gradually decreased the protein level of catalase in both fibroblast groups (Fig. 6 D). Catalase activity was reduced by TGF-β1 in control and IPF fibroblasts, but not in the same manner since IPF fibroblasts were less sensitive towards lower concentrations of TGF-β1 (2.5 and 5 ng/ml; Fig. 6 E). Activation of PPAR-γ increased the protein level of catalase in the absence of TGF-β1 (Additional file: Fig. S6K) and reversed the TGF-β1-induced decrease in catalase in control fibroblasts (Fig. 6 F). The level of catalase increased in both groups when PPAR-β/δ and PPAR-γ were activated 24 h after TGF-β1 treatment (Fig. 6 F), but not when added together with TGF-β1 (Fig. 6 G).
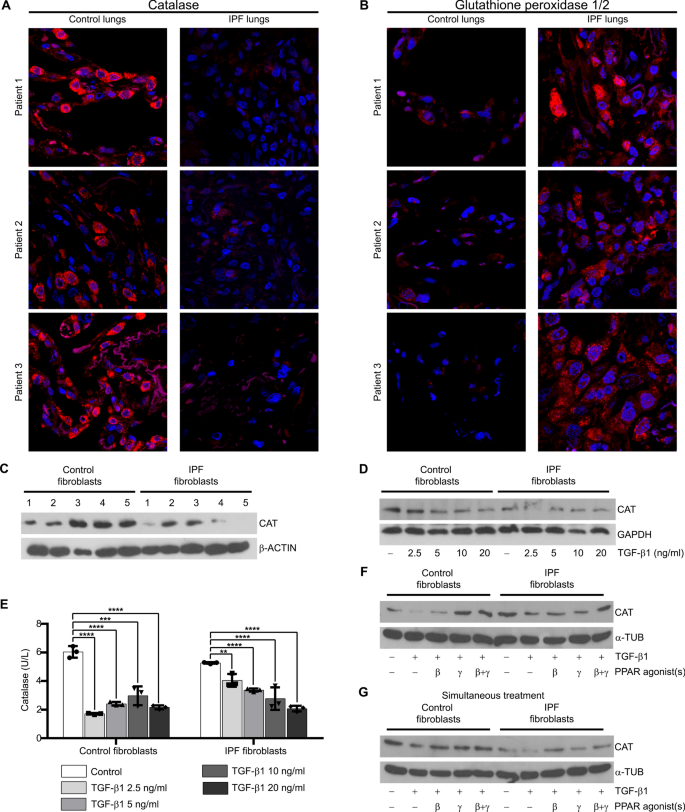
TGF-β1 caused a decrease in catalase mRNA and protein levels. A , B The immunoreactivity of catalase was lower, and that of GPX1/2 higher in IPF (right) compared to control (left) lung tissues. Immunofluorescence staining was performed using antibodies to detect catalase ( A , red) and GPX1/2 ( B , red) and DAPI to counterstain nuclei. C The protein level of catalase is lower in IPF than in control fibroblasts. Cell lysates of fibroblasts from 5 control and 5 IPF patients were used for Western blot analysis of catalase (CAT) with β-actin (β-ACTIN) as reference protein. D TGF-β1 decreased catalase protein levels in control and IPF fibroblasts. Cells were serum-starved for 3 h, and treated with various concentrations of TGF-β1 or vehicle for 48 h. Cell lysates were used for Western blot analysis of catalase with GAPDH as reference protein. E – G Activation of PPAR-β/δ in combination with PPAR-γ restored TGF-β1-induced decrease in catalase protein levels and activity. E TGF-β1 decreased catalase activity in control and IPF fibroblasts. Cells were serum-starved for 3 h, and treated with vehicle (Control) or various concentrations of TGF-β1 for 12 h. Cell lysates were used for measuring catalase activity. F , G Activation of PPAR-β/δ in combination with PPAR-γ inhibited TGF-β1-induced decrease in catalase protein levels in control and IPF fibroblasts. Cells were serum-starved for 3 h, stimulated with vehicle ( F , G ) or TGF-β1 (5 ng/ml, F , G ) or for 24 h, followed by the addition of the PPAR-β/δ agonist GW0742 (10 μM, β) and the PPAR-γ agonist rosiglitazone (10 μM, γ) for another 24 h ( F ). In ( G ), the PPAR agonists were added together with TGF-β1 for 48 h. Cell lysates were used to detect catalase (CAT) by Western blot analysis using α-tubulin (α-TUB) as reference protein
Catalase contributes to collagen reduction in pulmonary fibrosis
To confirm the anti-fibrotic role of catalase in IPF, we intended to generate stable catalase-deficient fibroblast cell lines by RNAi using two independent shRNAs against catalase (CAT sh1 RNA and CAT sh2 RNA). Knockdown efficiency of catalase was high and stable in control fibroblasts, whereas IPF fibroblasts died after a few passages probably because the catalase protein level was already low prior to shRNA transduction (see Fig. 6 C) and a further decrease in this protein was detrimental. Successful reduction of catalase is shown on the protein (Fig. 7 A) and activity (Fig. 7 B) levels, resulting in an increase in H 2 O 2 concentration (Fig. 7 C). The decrease in catalase protein in control fibroblasts expressing either of the two independent catalase shRNAs was accompanied with increased extracellular collagen (Fig. 7 D) and intracellular COL1 (Fig. 7 A) levels. Using siRNA technology, a transient catalase knockdown was achieved in control and IPF fibroblasts (Additional file: Fig. S7A). In IPF fibroblasts, we detected higher levels of collagen released into the culture medium compared to those transfected with scrambled control siRNA (Additional file: Fig. S7B). Moreover, catalase overexpression in control and IPF fibroblasts decreased COL1 and α-SMA protein levels even after TGF-β1 stimulation (Fig. 7 E). Lastly, we analyzed whether the reduction in collagen synthesis by activation of PPAR-β/δ and PPAR-γ depends on catalase activity. In both fibroblast cell lines, the reduction in collagen by the PPAR-γ agonist, but not by PPAR-β/δ was reversed in the presence of 3-amino-1,2,4-triazole (AT, Fig. 7 F, lane 5 versus lanes 7 and 8). Interestingly, AT inhibited the beneficial effect of a combined activation of PPAR-β/δ and PPAR-γ in control, but not in IPF fibroblasts (Fig. 7 F, lane 5 versus lane 6). We suggest that during TGF-β1 treatment either the protein level, sensitivity or signaling of PPAR-β/δ dominates in IPF and that of PPAR-γ in control fibroblasts with regard to catalase protein content and its activity.
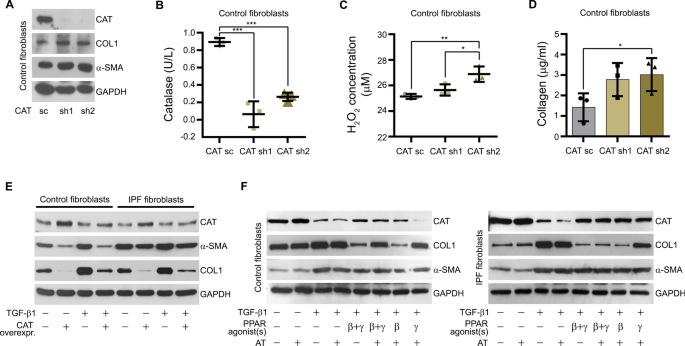
Catalase contributes to collagen reduction in pulmonary fibrosis. A , B Stable knockdown of catalase decreased catalase protein and activity. Cell lines transfected with catalase shRNA (CAT sh1, CAT sh2) were serum-starved for 3 h. Cell lysates were used for measuring catalase (CAT), COL1 and α-SMA protein levels by Western blot analysis using GAPDH as reference protein ( A ) and catalase activity by catalase activity assay kit ( B ). C , D Stable knockdown of catalase increased the cellular H 2 O 2 production and extracellular collagen levels. Culture media from catalase-deficient (CAT sh1, CAT sh2) and mock-transfected (CAT sc) control fibroblasts were used to detect the release of H 2 O 2 using the hydrogen peroxide assay ( C ) and of extracellular collagen by Sircol assay ( D ). E Overexpression of catalase decreased the protein level of COL1 in control and IPF fibroblasts under basal condition (no treatment) and after TGF-β1 treatment. Control and IPF fibroblasts were transfected with pGL 4.14-Catalase (CAT overexpr.) or a mock vector for 48 h, followed by the addition of vehicle or TGF-β1 (5 ng/ml) for another 48 h. Cell lysates were analyzed for catalase (CAT), α-SMA, and COL1 protein levels by Western blot analysis using GAPDH as reference protein. F The catalase activity inhibitor AT does not increase COL1 in control and IPF fibroblasts. Cells were serum-starved for 3 h, treated with vehicle or TGF-β1 (5 ng/ml) or for 24 h, followed by the addition of the PPAR-β/δ agonist GW0742 (10 μM, β), the PPAR-γ agonist rosiglitazone (10 μM, γ) and AT (25 µM) as well as various combinations thereof for another 24 h. Cell lysates were used to analyze catalase (CAT), COL1, and α-SMA protein levels by Western blot analysis using GAPDH as reference protein
In the present study, cultured human lung fibroblasts were treated with TGF-β1 to mimic fibrosis and were then analyzed to evaluate the role of PPARs during disease progression. Human lung tissue samples from control and IPF patients (Figs. 1 , 6 ) were used in parallel. Traditional animal models of experimental lung fibrosis were carried out by radiation or intratracheal administration of asbestosis fibers and silica, but the latter two induce rather asbestosis and silicosis than fibrosis [ 38 ]. Since high levels of TGF-β1 were shown to initiate and support fibrosis [ 35 , 36 ], a rat model of adenoviral overexpression of TGF-β1 has been established, however, the adenovirus vector itself already induced fibrosis [ 38 , 39 ]. Most commonly, mice were treated with bleomycin which induced a rapid fibrosis within 2–4 weeks via intra-tracheal instillation or 4–12 weeks by systemic administration [ 38 ]. The injury first triggers an inflammatory response which leads to wound healing. The infiltrating immune cells produce pro-fibrotic cytokines, e.g. TGF-β1, which stimulates fibroblast-to-myofibroblast transition. A dysregulated wound healing process could moreover lead to excessive deposition of ECM and finally resulting in fibrosis. However, this mouse model does not represent all aspects of the histopathological phenotype of the disease as observed in humans, for example, honeycomb pattern, thick scars at the alveolar region and fibroblastic foci [ 40 , 41 , 42 ], probably because these features take time to develop in humans. In addition, bleomycin-induced fibrosis is often reversible and contains a strong inflammatory component in the beginning which is not true for the disease in humans [ 38 ].
To mimic fibrosis in vitro, pro-fibrotic cytokines were added to cultured lung fibroblasts such as platelet-derived growth factor, connective tissue growth factor, interleukin-1β, tumor necrosis factor-α (TNF-α) and TGF-β1 [ 43 ]. Interleukin-1β and growth factors induced a marked inflammation and fibrosis with aberrant wound healing, TNF-α induced a strong inflammation and mild fibrosis, and TGF-β1 solely caused minor inflammation together with a marked fibrosis. Thus, TGF-β1-induced changes reflected the pathogenesis found in human IPF patients and was therefore used in our experiments. In vitro models, as an advantage, allow drug treatments to block TGF-β1-induced fibrosis signaling pathways and cell transfection to knockdown proteins of interest, which is difficult to establish in vivo. On the other hand, analysis of cultured lung fibroblasts neglects the in vivo situation where they interact with themselves and other cell types such as alveolar epithelial cells type I and type II, endothelial cells and macrophages. Interestingly, alveolar epithelial type II cells restrict the number of fibroblasts [ 44 ], and thus, control fibroblasts in vitro (and in the absence of alveolar epithelial type II cells) might re-start proliferation together with an increased collagen synthesis reaching similar levels as found in IPF fibroblasts. Moreover, TGF-β1 in IPF is mainly produced by macrophages [ 45 ]. Therefore, TGF-β1 (at least 5 ng/ml) had to be added to induce fibrosis in cultures of pure fibroblasts (which secrete 0.15 ng/ml TGF-β1, Fig. 1 C). In this study, tissues and an in vitro model established with fibroblasts from control and IPF patients were used in parallel.
To study the pathophysiology of lung fibrosis, we measured the two fibrosis markers associated with IPF such as collagen [ 46 , 47 , 48 ], and α-SMA, although the latter has been currently debated as a sole marker for studying fibrosis [ 49 ] as its expression doesn´t mean that a cell produces high amounts of collagen [ 50 ]. Interestingly, IPF is characterized by excessive accumulation of collagen-rich ECM produced by activated fibroblasts and myofibroblasts [ 51 , 52 ]; thus the degree of fibrosis is strongly dependent on their number and proliferation. Our data showed that fibroblasts from control and IPF patients were not different with regard to (1) the intracellular level of α-SMA and ω-fatty acids such as AA, DHA and EPA; (2) the release of collagen into the extracellular space; (3) the activity of collagen-degrading enzyme MMP-1; and (4) cell proliferation rate under basal conditions. Instead, fibroblasts from IPF compared to control patients showed significantly lower protein levels of PEX13, catalase, and of the TGFBR1 and are thus less sensitive towards TGF-β1. They secrete less active TGF-β1 into the culture medium. Contrarily, higher protein levels were found in IPF compared to control fibroblasts for intracellular GPX1/2 and PPAR-α. For IPF, the number and proliferation of fibroblasts/myofibroblasts are directly and the level of catalase indirectly related to the disease progression. Nonetheless, individual fibroblasts from control and IPF patients differ strongly even within the group (Figs. 1 D, 6 C , Additional file: Fig. S1A–C). This phenomenon might probably be due to the recently reported spatial heterogeneity of fibroblasts in fibrotic foci containing multiple subtypes such as lipofibroblasts, myofibroblasts, EBF1 + fibroblasts, intermediate fibroblasts, and mesothelial cells, all expressing different amounts of collagen under healthy conditions and during IPF progression [ 50 ]. In addition, the patients differ either with regard to the disease (acute exacerbation versus chronic stages, slow versus rapid decline of lung function), to co-morbidities (hypertension, viral infection, chronic aspiration of gastric content) or to other trigger factors such as age (age-related mitochondrial and peroxisomal dysfunction leading to oxidative stress), environmental exposures, smoking, and genetic factors [ 53 ]. Interestingly, differences between patients in our experiments were mainly observed for protein levels of PPAR-α (Fig. 1 D), PPAR-γ (Fig. 1 D), MMP-1 (Additional file: Fig. S1C) and catalase (Fig. 6 C), whereas the protein levels of PPAR-β/δ (Fig. 1 D), catalase activity (Fig. 6 E), the level of intracellular and secreted collagen with and without TGF-β1 (Figs. 1 B, 2 B–D, Additional file: Fig. S1D) as well as the collagen-reducing effect of a combined treatment with PPAR-β/δ and PPAR-γ agonists (Fig. 3 A–F) were less variable. This gives hope that the observed beneficial effect of PPAR-β/δ and PPAR-γ agonists is applicable to a broad spectrum of IPF patients. However, the strong heterogeneity of the target, namely the fibroblasts of IPF, but also of control patients, will limit the global use of any drug for IPF. Clinical trials discriminating between different subsets of patients may help to find the right drug in this regard.
We demonstrated that among the three PPARs, PPAR-β/δ might be a strong target for lung fibrosis resolution compared to PPAR-α (minor effect) and PPAR-γ (additive effect with PPAR-β/δ under these experimental conditions, Table 2 ). Focusing first on fibrosis pathways, we detected no differences between control and IPF fibroblasts with regard to the synthesis and release of collagen as well as gene expression and activity of MMP-1 (the dominant MMP, Fig. 4 A) either when treated or untreated with TGF-β1, and PPAR-β and PPAR-γ agonists . However, MMPs differ between the diverse lung cell types such as alveolar epithelial type I and type II cells, alveolar macrophages and endothelial cells [ 54 , 55 ]. In addition, MMP-1, -2, -3, -7, -13, -14, and -19, exhibit either anti- or pro-fibrotic [ 28 ] activities. MMP-2, as an example for the latter one, cleaves elastin which is deleterious for the lung. Interestingly, PPAR-β stimulation decreased the secretion of MMP-2 and increased the elastin level in human skin fibroblasts [ 56 ].
Next, we observed a TGF-β1-induced decrease in the peroxisomal biogenesis protein PEX13 which is reversed by stimulation of PPAR-γ. This was accompanied by changes in peroxisomal lipid metabolism, e.g. TGF-β1 increased the level of phosphatidylcholine in control, but decreased it in IPF fibroblasts with no additional effects of the PPAR drugs. The levels of AA, DHA and EPA were not significantly changed by TGF-β1, but increased strongly upon treatment with the PPAR-γ agonist. Metabolites from AA oxidation have been described to mediate inflammatory responses, and DHA is known to be anti-inflammatory [ 57 , 58 ]. A balance between the fatty acids will essentially determine the direction of the drug interventions. The production of DHA was more than that of AA in control and IPF fibroblasts following PPAR-γ activation, whereas the activation of PPAR-β/δ increased levels of AA to a higher extent compared to DHA in control and IPF fibroblasts. However, the strong anti-fibrotic effects of PPAR-β/δ support the combined activation of both receptors during treatments. Thus, with regard to peroxisomes, PPAR-β/δ and PPAR-γ agonists increased the peroxisomal biogenesis protein PEX13, as well as peroxisome lipid metabolism, and the resulting metabolites may further activate PPARs, establishing a positive activation loop [ 59 , 60 ].
Furthermore, the TGF-β1-induced decrease in the protein level and activity of catalase was reversed upon stimulation of PPAR-γ and PPAR-β/δ. Interestingly, in control fibroblasts the anti-fibrotic effect is mediated mainly via the maintenance of catalase protein through a reactive oxygen species (ROS)-dependent stimulation of PPAR-γ, because the effect is blocked by the specific catalase inhibitor AT in the combined treatment group by sustaining catalase levels. In IPF fibroblasts, the anti-fibrotic effect is mainly caused by a combined activation of PPAR-β/δ and PPAR-γ. The collagen-reducing effect is not inhibited by AT and thus ROS-independent. A decreased catalase level has been found in lung homogenates (and especially in the bronchial epithelium) of patients with IPF [ 61 ]. In acatalasemic mice, bleomycin induced a much higher invasion of pro-inflammatory cells together with increased levels of TGF-β1 and collagen and thus a higher degree of fibrosis [ 29 ], suggesting a beneficial role of high catalase levels in IPF disease progression. Interestingly, catalase (low affinity, high turnover) together with PRDX1 and PRDX5 (high affinity, low turnover), breakdown H 2 O 2 generated by multiple pathways inside peroxisomes. While catalase is crucial for safeguarding the organelle at excessive H 2 O 2 , PRDX1 and PRDX5 function as a redox-regulator in cell signaling and H 2 O 2 redox relay factor at low levels of H 2 O 2 , respectively [ 62 ]. In addition, catalase impedes ROS-induced inhibition of peroxisomal β-oxidation including the synthesis of the anti-inflammatory DHA [ 61 ]. With regard to PPARs, the catalase gene promotor region contains PPRE binding sites, e.g. for PPAR-γ (located at nucleotides − 1027 to − 1014; [ 63 ]) and an additional PPAR-γ binding site in humans only (located at nucleotides − 11,710 to − 11,698, [ 64 ]). Activation of PPAR-γ [ 23 ], but also of PPAR-β/δ (at the direct repeat 1 response element, [ 65 ]) increased catalase protein levels [ 65 , 66 ]. We assume that the observed increase in catalase protein in our experiments by PPAR-β/δ and PPAR-γ was similarly due to an induction of the catalase promotor activity. The additive effect by the combined treatment with PPAR-β/δ and PPAR-γ ligands in IPF fibroblasts suggests an importance of the additional human-specific PPRE binding sites and demonstrates that human models are required to analyze the role of PPARs in fibrosis.
We would like to emphasize that in contrast to most of the previous publications we performed a post-treatment (to mimic the clinical situation) with a combination of PPAR-β/δ and PPAR-γ agonists to reverse the TGF-β1-induced fibrotic phenotype of IPF fibroblasts. It is well known that activated PPAR-γ alone is potentially anti-fibrotic [ 17 , 18 , 19 , 20 ]. With regard to PPAR-β/δ, to the best of our knowledge, only one review described an inhibition of the proliferation of normal human lung fibroblasts by its stimulation [ 26 ]. The question arises how an activation of PPAR-β/δ can support PPAR-γ or vice-versa. One possibility is that stimulation of one PPAR might increase the protein level of itself and of the other receptors. For example, agonists for PPAR-α and PPAR-β/δ, but not PPAR-γ, have been shown to increase the protein levels of PPAR-β/δ and PPAR-γ in osteoblasts [ 37 ]. Thus, especially PPAR-β/δ stimulation can end up in a positive activation loop as it increased its own as well as the PPAR-γ receptor [ 60 ]. This offers the possibility for a post-treatment schedule starting with the PPAR-β/δ agonist to increase PPAR-γ levels so that the later given PPAR-γ agonist can work more efficiently. Interestingly, after 48 h treatment with TGF-β1, we observed increases in the protein levels of PPAR-γ and PPAR-β/δ in control and IPF fibroblasts although with varying degrees (Fig. 2 E, Table 1 ). This might explain why the post-treatment with PPAR-β/δ and PPAR-γ agonists is even more beneficial than direct treatment. Moreover, we demonstrated that the test compound STK 648389 (ZINC ID: 31,775,965), which has been suggested to be a dual PPAR-β/δ/PPAR-γ agonist by structure-based virtual screening [ 67 ], did not elicit anti-fibrotic effects (Additional file: Fig. S2). We hypothesized that the dual agonist (which is a single molecule) might be less specific for both receptors than the respective individual agonists and must be applied at a higher concentration which could induce more side effects in lung fibroblasts. Indeed, luciferase transactivation assays have shown EC50 values of 132 µM for PPAR-β/δ and 18 µM for PPAR-γ [ 67 ], and thus STK 648389 activated PPAR-γ only (see Fig. 3 E showing no reduction of the extracellular collagen using 10 µM of the specific PPARγ agonist troglitazone).
In summary, combined activation of PPAR-β/δ and PPAR-γ exerts strong anti-fibrotic effects. Catalase, which is decreased during treatment with TGF-β1, is inverse proportionally involved in collagen production. Catalase protein level and activity can be increased by stimulation of PPAR-β/δ and PPAR-γ in control and IPF human lung fibroblasts. For IPF patients (to refer to the clinical situation), the most beneficial anti-fibrotic effects could possibly be achieved by a combined local treatment with PPAR-β/δ and PPAR-γ agonists via aerosol inhalation.
Availability of data and materials
Raw data of the lipid analyses are available upon request to the corresponding author.
Abbreviations
α-Smooth muscle actin
Arachidonic acid
3-Amino-1,2,4-triazole
Collagen type 1
Dulbecco’s Modified Eagle Medium
Docosahexaenoic acid
Extracellular matrix
Eicosapentaenoic acid
Glutathione peroxidase 1/2
Horse radish peroxidase
- Idiopathic pulmonary fibrosis
Liquid chromatography tandem mass spectrometry
Matrix metalloproteinase
Methyl- tert -butyl ether
Phosphatidylcholine
Phosphatidylethanolamine
Peroxisomal biogenesis protein, peroxin
Peroxisome proliferator-activated receptor
Peroxiredoxin
PPAR response element
Reactive oxygen species
Quantitative reverse transcription polymerase chain reaction
Sphingomyelin
Solid phase extraction
Triglycerides
Transforming growth factor-beta 1
Transforming growth factor-beta receptor 1
Tumor necrosis factor-α
Maher TM. Interstitial lung disease: a review. JAMA. 2024;331:1655–65. https://doi.org/10.1001/jama.2024.3669 .
Article PubMed CAS Google Scholar
Cheresh P, Kim SJ, Tulasiram S, Kamp DW. Oxidative stress and pulmonary fibrosis. Biochim Biophys Acta. 2013;1832:1028–40. https://doi.org/10.1016/j.bbadis.2012.11.021 .
Richeldi L, du Bois RM, Raghu G, Azuma A, Brown KK, Costabel U, Cottin V, Flaherty KR, Hansell DM, Inoue Y, Kim DS, Kolb M, Nicholson AG, Noble PW, Selman M, Taniguchi H, Brun M, Le Maulf F, Girard M, Stowasser S, Schlenker-Herceg R, Disse B, Collard HR, INPULSIS Trial Investigators. Efficacy and safety of nintedanib in idiopathic pulmonary fibrosis. N Engl J Med. 2014;370:2071–82. https://doi.org/10.1056/NEJMoa1402584 .
Raghu G, Remy-Jardin M, Richeldi L, Thomson CC, Inoue Y, Johkoh T, Kreuter M, Lynch DA, Maher TM, Martinez FJ, Molina-Molina M, Myers JL, Nicholson AG, Ryerson CJ, Strek ME, Troy LK, Wijsenbeek M, Mammen MJ, Hossain T, Bissell BD, Herman DD, Hon SM, Kheir F, Khor YH, Macrea M, Antoniou KM, Bouros D, Buendia-Roldan I, Caro F, Crestani B, Ho L, Morisset J, Olson AL, Podolanczuk A, Poletti V, Selman M, Ewing T, Jones S, Knight SL, Ghazipura M, Wilson KC. Idiopathic pulmonary fibrosis (an update) and progressive pulmonary fibrosis in adults: an official ATS/ERS/JRS/ALAT clinical practice guideline. Am J Respir Crit Care Med. 2022;205:e18–47. https://doi.org/10.1164/rccm.202202-0399ST .
Article PubMed PubMed Central Google Scholar
King TE Jr, Bradford WZ, Castro-Bernardini S, Fagan EA, Glaspole I, Glassberg MK, Gorina E, Hopkins PM, Kardatzke D, Lancaster L, Lederer DJ, Nathan SD, Pereira CA, Sahn SA, Sussman R, Swigris JJ, Noble PW, ASCEND Study Group. A phase 3 trial of pirfenidone in patients with idiopathic pulmonary fibrosis. N Engl J Med. 2014;370:2083–92. https://doi.org/10.1056/NEJMoa1402582 .
Sofia C, Comes A, Sgalla G, Richeldi L. Promising advances in treatments for the management of idiopathic pulmonary fibrosis. Expert Opin Pharmacother. 2024;25:717–25. https://doi.org/10.1080/14656566.2024.2354460 .
Bonella F, Spagnolo P, Ryerson C. Current and future treatment landscape for idiopathic pulmonary fibrosis. Drugs. 2023;83:1581–93. https://doi.org/10.1007/s40265-023-01950-0 .
Richeldi L, Azuma A, Cottin V, Hesslinger C, Stowasser S, Valenzuela C, Wijsenbeek MS, Zoz DF, Voss F, Maher TM, 1305-0013 Trial Investigators. Trial of a preferential phosphodiesterase 4B inhibitor for idiopathic pulmonary fibrosis. N Engl J Med. 2022;386:2178–87. https://doi.org/10.1056/NEJMoa2201737 .
Waxman A, Restrepo-Jaramillo R, Thenappan T, Engel P, Bajwa A, Ravichandran A, Feldman J, Hajari Case A, Argula RG, Tapson V, Smith P, Deng C, Shen E, Nathan SD. Long-term inhaled treprostinil for pulmonary hypertension due to interstitial lung disease: INCREASE open-label extension study. Eur Respir J. 2023;61:2202414. https://doi.org/10.1183/13993003.02414-2022 .
Article PubMed PubMed Central CAS Google Scholar
Nathan SD, Behr J, Cottin V, Lancaster L, Smith P, Deng CQ, Pearce N, Bell H, Peterson L, Flaherty KR. Study design and rationale for the TETON phase 3, randomised, controlled clinical trials of inhaled treprostinil in the treatment of idiopathic pulmonary fibrosis. BMJ Open Respir Res. 2022;9: e001310. https://doi.org/10.1136/bmjresp-2022-001310 .
Toyama T, Nakamura H, Harano Y, Yamauchi N, Morita A, Kirishima T, Minami M, Itoh Y, Okanoue T. PPARalpha ligands activate antioxidant enzymes and suppress hepatic fibrosis in rats. Biochem Biophys Res Commun. 2004;324:697–704. https://doi.org/10.1016/j.bbrc.2004.09.110 .
Ogata T, Miyauchi T, Sakai S, Irukayama-Tomobe Y, Goto K, Yamaguchi I. Stimulation of peroxisome-proliferator-activated receptor alpha (PPAR alpha) attenuates cardiac fibrosis and endothelin-1 production in pressure-overloaded rat hearts. Clin Sci (Lond). 2002;103:S284–8. https://doi.org/10.1042/CS103S284S .
Article Google Scholar
Oruqaj G, Karnati S, Vijayan V, Kotarkonda LK, Boateng E, Zhang W, Ruppert C, Günther A, Shi W, Baumgart-Vogt E. Compromised peroxisomes in idiopathic pulmonary fibrosis, a vicious cycle inducing a higher fibrotic response via TGF-beta signaling. Proc Natl Acad Sci USA. 2015;112:2048–57. https://doi.org/10.1073/pnas.1415111112 .
Article CAS Google Scholar
Oruqaj G, Karnati S, Kotarkonda LK, Boateng E, Bartkuhn M, Zhang W, Ruppert C, Günther A, Bartholin L, Shi W, Baumgart-Vogt E. Transforming growth factor-b1 regulates peroxisomal genes/proteins via Smad signaling in idiopathic pulmonary fibrosis fibroblasts and transgenic mouse models. Am J Pathol. 2023;193:259–74. https://doi.org/10.1016/j.ajpath.2022.11.006 .
Foreman JE, Sharma AK, Amin S, Gonzalez FJ, Peters JM. Ligand activation of peroxisome proliferator-activated receptor-beta/delta (PPARbeta/delta) inhibits cell growth in a mouse mammary gland cancer cell line. Cancer Lett. 2010;288:219–25. https://doi.org/10.1016/j.canlet.2009.07.006 .
Ham SA, Kim HJ, Kim HJ, Kang ES, Eun SY, Kim GH, Park MH, Woo IS, Kim HJ, Chang KC, Lee JH, Seo HG. PPARdelta promotes wound healing by up-regulating TGF-beta1-dependent or -independent expression of extracellular matrix proteins. J Cell Mol Med. 2010;14:1747–59. https://doi.org/10.1111/j.1582-4934.2009.00816.x .
Burgess HA, Daugherty LE, Thatcher TH, Lakatos HF, Ray DM, Redonnet M, Phipps RP, Sime PJ. PPARgamma agonists inhibit TGF-beta induced pulmonary myofibroblast differentiation and collagen production: implications for therapy of lung fibrosis. Am J Physiol Lung Cell Mol Physiol. 2005;288:L1146–53. https://doi.org/10.1152/ajplung.00383.2004 .
Milam JE, Keshamouni VG, Phan SH, Hu B, Gangireddy SR, Hogaboam CM, Standiford TJ, Thannickal VJ. Reddy RC PPAR-gamma agonists inhibit profibrotic phenotypes in human lung fibroblasts and bleomycin-induced pulmonary fibrosis. Am J Physiol Lung Cell Mol Physiol. 2008;294:L891–901. https://doi.org/10.1152/ajplung.00333.2007 .
Ferguson HE, Kulkarni A, Lehmann GM, Garcia-Bates TM, Thatcher TH, Huxlin KR, Phipps RP, Sime PJ. Electrophilic peroxisome proliferator-activated receptor-gamma ligands have potent antifibrotic effects in human lung fibroblasts. Am J Respir Cell Mol Biol. 2009;41:722–30. https://doi.org/10.1165/rcmb.2009-0006OC .
Lin Q, Fang LP, Zhou WW, Liu XM. Rosiglitazone inhibits migration, proliferation, and phenotypic differentiation in cultured human lung fibroblasts. Exp Lung Res. 2010;36:120–8. https://doi.org/10.3109/01902140903214659 .
El Agha E, Moiseenko A, Kheirollahi V, De Langhe S, Crnkovic S, Kwapiszewska G, Szibor M, Kosanovic D, Schwind F, Schermuly RT, Henneke I, MacKenzie B, Quantius J, Herold S, Ntokou A, Ahlbrecht K, Braun T, Morty RE, Günther A, Seeger W, Bellusci S. Two-way conversion between lipogenic and myogenic fibroblastic phenotypes marks the progression and resolution of lung fibrosis. Cell Stem Cell. 2017;20:261-73.e3. https://doi.org/10.1016/j.stem.2016.10.004 .
Speca S, Dubuquoy C, Rousseaux C, Chavatte P, Desreumaux P, Spagnolo P. GED-0507 attenuates lung fibrosis by counteracting myofibroblast transdifferentiation in vivo and in vitro. PLoS ONE. 2021;16: e0257281. https://doi.org/10.1371/journal.pone.0257281 .
Korbecki J, Bobiński R, Dutka M. Self-regulation of the inflammatory response by peroxisome proliferator-activated receptors. Inflamm Res. 2019;68:443–58. https://doi.org/10.1007/s00011-019-01231-1 .
Derrett-Smith E, Clark KEN, Shiwen X, Abraham DJ, Hoyles RK, Lacombe O, Broqua P, Junien JL, Konstantinova I, Ong VH, Denton CP. The pan-PPAR agonist lanifibranor reduces development of lung fibrosis and attenuates cardiorespiratory manifestations in a transgenic mouse model of systemic sclerosis. Arthritis Res Ther. 2021;23:234. https://doi.org/10.1186/s13075-021-02592-x .
Avouac J, Konstantinova I, Guignabert C, Pezet S, Sadoine J, Guilbert T, Cauvet A, Tu L, Luccarini JM, Junien JL, Broqua P, Allanore Y. Pan-PPAR agonist IVA337 is effective in experimental lung fibrosis and pulmonary hypertension. Ann Rheum Dis. 2017;76:1931–40. https://doi.org/10.1136/annrheumdis-2016-210821 .
Lakatos HF, Thatcher TH, Kottmann RM, Garcia TM, Phipps RP, Sime PJ. The role of PPARs in lung fibrosis. PPAR Res. 2007;2007:71323. https://doi.org/10.1155/2007/71323 .
Zeng Q, Zhou TT, Huang WJ, Huang XT, Huang L, Zhang XH, Sang XX, Luo YY, Tian YM, Wu B, Liu L, Luo ZQ, He B, Liu W, Tang SY. Asarinin attenuates bleomycin-induced pulmonary fibrosis by activating PPARgamma. Sci Rep. 2023;13:14706. https://doi.org/10.1038/s41598-023-41933-5 .
Craig VJ, Zhang L, Hagood JS, Owen CA. Matrix metalloproteinases as therapeutic targets for idiopathic pulmonary fibrosis. Am J Respir Cell Mol Biol. 2015;53:585–600. https://doi.org/10.1165/rcmb.2015-0020TR .
Odajima N, Betsuyaku T, Nagai K, Moriyama C, Wang DH, Takigawa T, Ogino K, Nishimura M. The role of catalase in pulmonary fibrosis. Respir Res. 2010;11:183. https://doi.org/10.1186/1465-9921-11-183 .
Smejkal GB, Kakumanu S. Enzymes and their turnover numbers. Expert Rev Proteomics. 2019;16:543–4. https://doi.org/10.1080/14789450.2019.1630275 .
Filatova A, Seidel S, Böğürcü N, Gräf S, Garvalov BK, Acker T. Acidosis acts through HSP90 in a PHD/VHL-independent manner to promote HIF function and stem cell maintenance in glioma. Cancer Res. 2016;76:5845–56. https://doi.org/10.1158/0008-5472.CAN-15-2630 .
Vijayan V, Srinu T, Karnati S, Garikapati V, Linke M, Kamalyan L, Mali SR, Sudan K, Kollas A, Schmid T, Schulz S, Spengler B, Weichhart T, Immenschuh S, Baumgart-Vogt E. A new immunomodulatory role for peroxisomes in macrophages activated by the TLR4 ligand lipopolysaccharide. J Immunol. 2017;198:2414–25. https://doi.org/10.4049/jimmunol.1601596 .
Matyash V, Liebisch G, Kurzchalia TV, Shevchenko A, Schwudke D. Lipid extraction by methyl-tert-butyl ether for high-throughput lipidomics. J Lipid Res. 2008;49:1137–46. https://doi.org/10.1194/jlr.D700041-JLR200 .
Garikapati V, Colasante C, Baumgart-Vogt E, Spengler B. Sequential lipidomic, metabolomic, and proteomic analyses of serum, liver, and heart tissue specimens from peroxisomal biogenesis factor 11a knockout mice. Anal Bioanal Chem. 2022;414:2235–50. https://doi.org/10.1007/s00216-021-03860-0 .
Khalil N, O’Connor RN, Unruh HW, Warren PW, Flanders KC, Kemp A, Bereznay OH, Greenberg AH. Increased production and immunohistochemical localization of transforming growth factor-beta in idiopathic pulmonary fibrosis. Am J Respir Cell Mol Biol. 1991;5:155–62. https://doi.org/10.1165/ajrcmb/5.2.155 .
Bergeron A, Soler P, Kambouchner M, Loiseau P, Milleron B, Valeyre D, Hance AJ, Tazi A. Cytokine profiles in idiopathic pulmonary fibrosis suggest an important role for TGF-beta and IL-10. Eur Respir J. 2003;22:69–76. https://doi.org/10.1183/09031936.03.00014703 .
Qian G, Fan W, Ahlemeyer B, Karnati S, Baumgart-Vogt E. Peroxisomes in different skeletal cell types during intramembranous and endochondral ossification and their regulation during osteoblast differentiation by distinct peroxisome proliferator-activated receptors. PLoS ONE. 2015;10: e0143439. https://doi.org/10.1371/journal.pone.0143439 .
Moore BB, Lawson WE, Tim Oury TD, Sisson TH, Raghavendran K, Hogaboam CM. Animal models of fibrotic lung disease. Am J Respir Cell Mol Biol. 2013;49:167–79. https://doi.org/10.1165/rcmb.2013-0094TR .
Sime PJ, Xing Z, Graham FL, Csaky KG, Gauldie J. Adenovector-mediated gene transfer of active transforming growth factor-beta1 induces prolonged severe fibrosis in rat lung. J Clin Invest. 1997;100:768–76. https://doi.org/10.1172/JCI119590 .
Fukuda Y, Basset F, Ferrans VJ, Yamanaka N. Significance of early intra-alveolar fibrotic lesions and integrin expression in lung biopsy specimens from patients with idiopathic pulmonary fibrosis. Hum Pathol. 1995;26:53–61. https://doi.org/10.1016/0046-8177(95)90114-0 .
Cavazza A, Rossi G, Carbonelli C, Spaggiari L, Paci M, Roggeri A. The role of histology in idiopathic pulmonary fibrosis: an update. Respir Med. 2010;104:S11-22. https://doi.org/10.1016/j.rmed.2010.03.013 .
Article PubMed Google Scholar
Jones MG, et al. Three-dimensional characterization of fibroblast foci in idiopathic pulmonary fibrosis. JCI Insight. 2016;1: e86375. https://doi.org/10.1172/jci.insight.86375 .
Kelly M, Kolb M, Bonniaud P, Gauldie J. Re-evaluation of fibrogenic cytokines in lung fibrosis. Curr Pharm Des. 2003;9:39–49. https://doi.org/10.2174/1381612033392341 .
Cerny L, Torday JS, Rehan VK. Prevention and treatment of bronchopulmonary dysplasia: contemporary status and future outlook. Lung. 2008;186:75–89. https://doi.org/10.1513/pats.201203-023AW .
Fernandez IE, Eickelberg O. The impact of TGF-beta on lung fibrosis: from targeting to biomarkers. Proc Am Thorac Soc. 2012;9:111–6. https://doi.org/10.1513/pats.201203-023AW .
Nakos G, Adams A, Andriopoulos N. Antibodies to collagen in patients with idiopathic pulmonary fibrosis. Chest. 1993;103:1051–8. https://doi.org/10.1378/chest.103.4.1051 .
Ramos C, Montaño M, García-Alvarez J, Ruiz V, Uhal BD, Selman M, Pardo A. Fibroblasts from idiopathic pulmonary fibrosis and normal lungs differ in growth rate, apoptosis, and tissue inhibitor of metalloproteinases expression. Am J Respir Cell Mol Biol. 2001;24:591–8. https://doi.org/10.1165/ajrcmb.24.5.4333 .
Su Y, Gu H, Weng D, Zhou Y, Li Q, Zhang F, Zhang Y, Shen L, Hu Y, Li H. Association of serum levels of laminin, type IV collagen, procollagen III N-terminal peptide, and hyaluronic acid with the progression of interstitial lung disease. Medicine (Baltimore). 2017;96: e6617. https://doi.org/10.1097/MD.0000000000006617 .
Sun KH, Chang Y, Reed NI, Sheppard D. alpha-Smooth muscle actin is an inconsistent marker of fibroblasts responsible for force-dependent TGFbeta activation or collagen production across multiple models of organ fibrosis. Am J Physiol Lung Cell Mol Physiol. 2016;310:L824–36. https://doi.org/10.1152/ajplung.00350.2015 .
Liu X, Dai K, Zhang X, Huang G, Lynn H, Rabata A, Liang J, Noble PW, Jiang D. Multiple fibroblast subtypes contribute to matrix deposition in pulmonary fibrosis. Am J Respir Cell Mol Biol. 2023;69:45–56. https://doi.org/10.1165/rcmb.2022-0292OC .
Zhang K, Rekhter MD, Gordon D, Phan SH. Myofibroblasts and their role in lung collagen gene expression during pulmonary fibrosis. A combined immunohistochemical and in situ hybridization study. Am J Pathol. 1994;145:114–25.
PubMed PubMed Central CAS Google Scholar
Zhang B, Berger J, Zhou G, Elbrecht A, Biswas S, White-Carrington S, Szalkowski D, Moller DE. Insulin- and mitogen-activated protein kinase-mediated phosphorylation and activation of peroxisome proliferator-activated receptor gamma. J Biol Chem. 1996;271:31771–4. https://doi.org/10.1074/jbc.271.50.31771 .
Gandhi S, Tonelli R, Murray M, Samarelli AV, Spagnolo P. Environmental causes of idiopathic pulmonary fibrosis. Int J Mol Sci. 2023;24:16481. https://doi.org/10.3390/ijms242216481 .
Wallace AM, Sandford AJ, English JC, Burkett KM, Li H, Finley RJ, Müller NL, Coxson HO, Paré PD, Abboud RT. Matrix metalloproteinase expression by human alveolar macrophages in relation to emphysema. COPD. 2008;5:13–23. https://doi.org/10.1080/15412550701817789 .
Garcia-de-Alba C, Buendia-Roldán I, Salgado A, Becerril C, Ramírez R, González Y, Checa M, Navarro C, Ruiz V, Pardo A, Selman M. Expression of matrix metalloproteases by fibrocytes: possible role in migration and homing. Am J Respir Crit Care Med. 2010;182:1144–52. https://doi.org/10.1164/rccm.201001-0028OC .
Ham SA, Yoo T, Hwang JS, Kang ES, Lee WJ, Paek KS, Park C, Kim JH, Do JT, Lim DS, Seo HG. Ligand-activated PPARδ modulates the migration and invasion of melanoma cells by regulating Snail expression. Am J Cancer Res. 2014;4:674–82.
PubMed PubMed Central Google Scholar
Tallima H, El Ridi R. Arachidonic acid: physiological roles and potential health benefits—a review. J Adv Res. 2018;11:33–41. https://doi.org/10.1016/j.jare.2017.11.004 .
Meital LT, Windsor MT, Perissiou M, Schulze K, Magee R, Kuballa A, Golledge J, Bailey TG, Askew CD, Russell FD. Omega-3 fatty acids decrease oxidative stress and inflammation in macrophages from patients with small abdominal aortic aneurysm. Sci Rep. 2019;9:12978. https://doi.org/10.1038/s41598-019-49362-z .
Kliewer SA, Forman BM, Blumberg B, Ong ES, Borgmeyer U, Mangelsdorf DJ, Umesono K, Evans RM. Fatty acids and eicosanoids regulate gene expression through direct interactions with peroxisome proliferator-activated receptors alpha and gamma. Proc Natl Acad Sci USA. 1997;94:4318–23. https://doi.org/10.1073/pnas.94.9.4318 .
Colasante C, Chen J, Ahlemeyer B, Baumgart-Vogt E. Peroxisomes in cardiomyocytes and the peroxisome/peroxisome proliferator-activated receptor-loop. Thromb Haemost. 2015;113:452–63. https://doi.org/10.1160/TH14-06-0497 .
Hashimoto F, Hayashi H. Significance of catalase in peroxisomal fatty acyl-CoA beta-oxidation: NADH oxidation by acetoacetyl-CoA and H 2 O 2 . J Biochem. 1990;108:426–31. https://doi.org/10.1093/oxfordjournals.jbchem.a123217 .
Fransen M, Lismont C. Peroxisomal hydrogen peroxide signalling: a new chapter in intracellular communication research. Curr Opin Chem Biol. 2024;78:102426. https://doi.org/10.1016/j.cbpa.2024.102426 .
Girnun GD, Domann FE, Moore SA, Robbins ME. Identification of a functional peroxisome proliferator-activated receptor response element in the rat catalase promoter. Mol Endocrinol. 2002;16:2793–801. https://doi.org/10.1210/me.2002-0020 .
Okuno Y, Matsuda M, Miyata Y, Fukuhara A, Komuro R, Shimabukuro M, Shimomura I. Human catalase gene is regulated by peroxisome proliferator activated receptor-gamma through a response element distinct from that of mouse. Endocr J. 2010;57:303–9. https://doi.org/10.1507/endocrj.k09e-113 .
Hur J, Kang ES, Hwang JS, Lee WJ, Won JP, Lee HG, Kim E, Seo HG. Peroxisome proliferator-activated receptor-delta-mediated upregulation of catalase helps to reduce ultraviolet B-induced cellular injury in dermal fibroblasts. J Dermatol Sci. 2021;103:167–75. https://doi.org/10.1016/j.jdermsci.2021.08.003 .
Hwang JS, Kim E, Lee HG, Lee WJ, Won JP, Hur J, Fujii J, Seo HG. Peroxisome proliferator-activated receptor δ rescues xCT-deficient cells from ferroptosis by targeting peroxisomes. Biomed Pharmacother. 2021;143: 112223. https://doi.org/10.1016/j.biopha.2021.112223 .
Maltarollo VG, Togashi M, Nascimento AS, Honorio KM. Structure-based virtual screening and discovery of new PPARd/g dual agonist and PPARd and g agonists. PLoS ONE. 2015;10: e0118790. https://doi.org/10.1371/journal.pone.0118790 .
Download references
Acknowledgements
Many thanks to Petra Hahn-Kohlberger, Bianca Pfeiffer, Andrea Textor and Susanne Pfreimer for their outstanding technical assistance. Sincere gratitude to Dr. Eunsum Jung (Biospectrum Life Science Institute) and Prof. Marc Fransen (Université catholique de Louvain, Belgium) for providing the COL1A2 luciferase and catalase overexpression plasmids, respectively.
Open Access funding enabled and organized by Projekt DEAL. This work was supported by funding from the German Academic Exchange Service (Government of Ghana DAAD, grant number 50015294) to EB, collaborative grant of the German Academic Exchange Service (DAAD) for granting the Graduate School Scholarship Programme "Lipids in Nutrition and Metabolism" (DAAD-GSSP-2015) to EBV, VG is a doctoral scholarship holder within this programme (Personal reference no 91566181), and performance-related resource allocation-funds of the Medical Faculty of the Justus Liebig University Giessen, so-called “ L eistungs o rientierte M ittel” (LOM) to EBV.
Author information
Srikanth Karnati
Present address: Institute for Anatomy and Cell Biology, Julius Maximilians University, 97070, Würzburg, Germany
Eistine Boateng
Present address: Department of Medical Education, College of Medicine and Life Sciences, University of Toledo, Toledo, OH, 43614, USA
Vannuruswamy Garikapati
Present address: Max Planck Institute of Molecular Cell Biology and Genetics, 01307, Dresden, Germany
Gani Oruqaj
Present address: Department of Internal Medicine II, Member of the German Center for Lung Research (DZL), Universities of Giessen and Marburg Lung Center (UGMLC), Justus Liebig University, 35392, Giessen, Germany
Natalia El-Merhie
Present address: Institute for Lung Health (ILH), Member of the German Center for Lung Research (DZL), Universities of Giessen and Marburg Lung Center (UGMLC), Justus Liebig University, 35392, Giessen, Germany
Srikanth Karnati and Eveline Baumgart-Vogt share senior authorship.
Authors and Affiliations
Institute for Anatomy and Cell Biology, Division of Medical Cell Biology, Justus Liebig University, Aulweg 123, 35392, Giessen, Germany
Eistine Boateng, Rocio Bonilla-Martinez, Barbara Ahlemeyer, Vannuruswamy Garikapati, Mohammad Rashedul Alam, Gani Oruqaj, Natalia El-Merhie, Srikanth Karnati & Eveline Baumgart-Vogt
Department of Internal Medicine VIII, Eberhard Karls University, 72076, Tübingen, Germany
Omelyan Trompak
Excellence Cluster Cardio-Pulmonary System, German Center for Lung Research (DZL), Universities of Giessen and Marburg Lung Center, 35392, Giessen, Germany
Michael Seimetz, Clemens Ruppert & Andreas Günther
UGMLC Giessen Biobank, Universities of Giessen and Marburg Lung Center, 35392, Giessen, Germany
Clemens Ruppert
Center for Interstitial and Rare Lung Diseases, Department of Internal Medicine, German Center for Lung Research, Universities of Giessen and Marburg Lung Center, 35392, Giessen, Germany
Andreas Günther
Institute of Inorganic and Analytical Chemistry, Justus Liebig University, 35392, Giessen, Germany
Vannuruswamy Garikapati & Bernhard Spengler
You can also search for this author in PubMed Google Scholar
Contributions
E.B.-V., S.K. and E.B. conceived and designed the research studies; E.B., R.B, B.A., O.T., V.G. and M.R.A. conducted the experiments; E.B.-V., B.A., B.S., S.K., R.B., V.G., N.E-M. and E.B. acquired and analyzed the data analyzed; E.B.-V., S.K., M.S., B.S., C.R., G.O., and A.G. provided reagents and materials; and E.B.-V., B.A., R.B. and E.B. wrote the manuscript. All authors reviewed the manuscript.
Corresponding author
Correspondence to Eveline Baumgart-Vogt .
Ethics declarations
Ethics approval and consent to participate.
Protocol, data collection and analysis of the human material (isolated lung fibroblasts, lung tissues) were approved by the Local Ethics Committee of the Justus Liebig University Giessen (Az58/15 and Az111/08, JLU).
Consent for publication
Not applicable.
Competing interests
The authors declare no competing interests.
Additional information
Publisher's note.
Springer Nature remains neutral with regard to jurisdictional claims in published maps and institutional affiliations.
Eistine Boateng, Vannuruswamy Garikapati, Gani Oruqaj, Natalia El-Merhie, Srikanth Karnati: All experimental work has been done at Institute for Anatomy and Cell Biology, Division of Medical Cell Biology, Justus Liebig University, Aulweg 123, 35392, Giessen, Germany. Vannuruswamy Garikapati: The experimental work has been done in a cooperative project at two places at the JLU Giessen (Institute for Anatomy and Cell Biology, Division of Medical Cell Biology, Justus Liebig University and Institute of Inorganic and Analytical Chemistry, Justus Liebig University).
Supplementary Information
Detailed description of the methods, 8 additional files., rights and permissions.
Open Access This article is licensed under a Creative Commons Attribution 4.0 International License, which permits use, sharing, adaptation, distribution and reproduction in any medium or format, as long as you give appropriate credit to the original author(s) and the source, provide a link to the Creative Commons licence, and indicate if changes were made. The images or other third party material in this article are included in the article's Creative Commons licence, unless indicated otherwise in a credit line to the material. If material is not included in the article's Creative Commons licence and your intended use is not permitted by statutory regulation or exceeds the permitted use, you will need to obtain permission directly from the copyright holder. To view a copy of this licence, visit http://creativecommons.org/licenses/by/4.0/ . The Creative Commons Public Domain Dedication waiver ( http://creativecommons.org/publicdomain/zero/1.0/ ) applies to the data made available in this article, unless otherwise stated in a credit line to the data.
Reprints and permissions
About this article
Cite this article.
Boateng, E., Bonilla-Martinez, R., Ahlemeyer, B. et al. It takes two peroxisome proliferator-activated receptors (PPAR-β/δ and PPAR-γ) to tango idiopathic pulmonary fibrosis. Respir Res 25 , 345 (2024). https://doi.org/10.1186/s12931-024-02935-7
Download citation
Received : 03 May 2024
Accepted : 01 August 2024
Published : 23 September 2024
DOI : https://doi.org/10.1186/s12931-024-02935-7
Share this article
Anyone you share the following link with will be able to read this content:
Sorry, a shareable link is not currently available for this article.
Provided by the Springer Nature SharedIt content-sharing initiative
- Human lung fibroblasts
- Matrix metalloproteinases
Respiratory Research
ISSN: 1465-993X
- General enquiries: [email protected]

IMAGES
VIDEO
COMMENTS
Class practical or demonstration. Hydrogen peroxide (H 2 O 2) is a by-product of respiration and is made in all living cells. Hydrogen peroxide is harmful and must be removed as soon as it is produced in the cell. Cells make the enzyme catalase to remove hydrogen peroxide.. This investigation looks at the rate of oxygen production by the catalase in pureed potato as the concentration of ...
In this catalase and hydrogen peroxide experiment, we will discover how enzymes act as catalysts by causing chemical reactions to occur more quickly within living things. Using a potato and hydrogen peroxide, we can observe how enzymes like catalase work to perform decomposition, or the breaking down, of other substances. Catalase works to ...
The enzyme catalase can speed up (catalyse) this reaction. In this practical, students investigate the presence of enzymes in liver, potato and celery by detecting the oxygen gas produced when hydrogen peroxide decomposes. The experiment should take no more than 20-30 minutes.
If there is no hydrogen peroxide present, the catalase cannot function, which is why in cup 1 you should not have seen any bubble or foam production. Only when hydrogen peroxide is available, the catalase reaction can take place as you probably observed in the other cups. In fact, the catalase reaction is dependent on the substrate concentration.
Figure 1. When blended liver is exposed to hydrogen peroxide, the catalase enzyme in the liver reacts with the hydrogen peroxide to form oxygen gas, which creates the visible bubbles in this photograph, and water. In this science fair project, you will use fresh (or frozen) liver as a source of catalase and investigate how the activity of the ...
Peroxide splits into a molecule of water and a molecule of oxygen, which causes the bubbles. In a series of tests, students observe how the enzyme catalase breaks down hydrogen peroxide into oxygen and water. Simply place a few drops of peroxide onto tissue and the bubbling will indicate the presence of the enzyme, catalase.
Then, they drop the spheres into hydrogen peroxide and measure how quickly they float to the surface. The time it takes to reach the surface is an indirect measure of the speed of the reaction. Students observe the spheres in different concentrations of hydrogen peroxide (3%, 1.5%, and .75%).
Objectives. In this experiment, you will. Use a Gas Pressure Sensor to measure the production of oxygen gas as hydrogen peroxide is destroyed by the enzyme catalase or peroxidase at various enzyme concentrations. Measure and compare the initial rates of reaction for this enzyme when different concentrations of enzyme react with H 2 O 2. Measure ...
In this Preliminary Activity, you will use catalase in yeast to catalytically decompose hydrogen peroxide. You will use an O 2 Gas Sensor to determine the rate of catalase activity by measuring oxygen gas produced as H 2 O 2 is decomposed.. At the start of the reaction, there is no product, and the O 2 concentration is the same as the atmosphere. Shortly after data collection begins, oxygen ...
The primary reaction catalyzed by catalase is the decomposition of hydrogen peroxide to form water and oxygen. 2H2O2 ——-> 2 H2O + O2 (gas) Without the help of catalase, this reaction occurs spontaneously, but very slowly. Catalase helps to speed up the reaction considerably. In this lab, a rate for this reaction will be determined.
In this experiment you are measuring the rate at which oxygen is formed from the enzyme catalase decomposing hydrogen peroxide. Here, the product oxygen gas, provides the means of following the rate of the reaction. Enzyme reaction equation: hydrogen peroxide === catalase ===> water + oxygen (gas)
Catalase speeds up the following reaction: 2 H 2 O 2-> 2 H 2 O + O 2. Hydrogen peroxide is toxic. Cells therefore use catalase to protect themselves. In these experiments, we will use catalase enzyme from potato. The first experiment will establish that our catalase works (positive control) and that our reagents are not contaminated (negative ...
Catalase. The structure and function of enzymes is a central theme in cellular and molecular biology. In this laboratory exercise, a crude cell extract is prepared from potatoes. Activity of the enzyme, catalase [which catalyzes the reaction 2H 2 O 2 (l) → 2H 2 O (l) + O 2 (g)], is then studied using a simple assay for O 2. To conduct the ...
Dropping paper discs soaked in the enzyme catalase into hydrogen peroxide solution and timing how log to sink then rise to the surface
In this experiment, you will Use an Oxygen Gas Sensor to measure the production of oxygen gas as hydrogen peroxide is destroyed by the enzyme catalase or peroxidase at various enzyme concentrations. Measure and compare the initial rates of reaction for this enzyme when different concentrations of enzyme react with H 2 O 2.
In this lab, you will study an enzyme that is found in the cells of many living tissues. The name of the enzyme is catalase; it speeds up a reaction which breaks down hydrogen peroxide, a toxic chemical, into 2 harmless substances--water and oxygen. Light can also break down H 2 O 2 which is why the chemical is sold in dark containers.
Spanish. Past Papers. CIE. Spanish Language & Literature. Past Papers. Other Subjects. Revision notes on 1.4.4 Required Practical: Measuring Enzyme Activity for the AQA A Level Biology syllabus, written by the Biology experts at Save My Exams.
Students who complete the Enzyme Investigation lab can further explore enzymes with this lab on how concentrations of the substrate (hydrogen peroxide) and the enzyme ( catalase) can affect the rate of reaction. In the first experiment, students simply made a judgement about the amount of bubbling to indicate reaction speed, though this is a ...
A beaker or bottle for the experiment; A bowl (to mix ingredients) A tablespoon; Tray; How to carry out the Elephant Toothpaste Experiment: Place the bottle for the experiment on the tray. (The tray will catch the foam created by the experiment.) Fill ⅓ of the bottle with hydrogen peroxide. Add a large squirt of dish soap to the bottle.
The improper disposal of uranium mine tailings and smelting wastewater poses significant environmental hazards, including contamination of water bodies and soil, and potential health risks to humans through the accumulation of uranium in bones and organs. This study examines the removal of hexavalent uranium (U(VI)) from water using biochar derived from agricultural waste enhanced by hydrogen ...
Hydrogen peroxide (H 2 O 2) is a common but poisonous by-product of cellular metabolism, but H 2 O 2 does not accumulate in cells because it is decomposed to water and oxygen gas. The decomposition of the hydrogen peroxide is facilitated by catalase, an enzyme present in most cells. The reaction is: 2H 2 O 2 → 2H 2 O + O 2
Objectives. In this Preliminary Activity, you will use catalase in yeast to catalytically decompose hydrogen peroxide. You will use an O 2 Gas Sensor to determine the rate of catalase activity by measuring oxygen gas produced as H 2 O 2 is decomposed. Before data collection begins, there is no product, and the pressure is the same as ...
Measurements of catalase activity, hydrogen peroxide (H 2 O 2) production and cell proliferation. Determination of catalase activity with a redox dye assay kit based on the degradation of H 2 O 2. H 2 O 2 produced by cultured cells was quantified using a fluorometric detection kit. The incorporation of BrdU into proliferating cells was detected ...